Fig. 11.1
Proangiogenic hematopoietic cells may interact with resident endothelial progenitor cells to promote neoangiogenesis. Circulating hematopoietic (bone marrow-derived) cells are proposed to provide paracrine signals that stimulate resident endothelial cell colony-forming cells to undergo neoangiogenesis. Schematic adapted from Basile and Yoder (2014)
Questions remain as to the location (niche) of resident endothelial colony-forming cells within the vessel wall, and how these cells interpret tissue-specific cues to control development, neo-angiogenesis, and the response to injury. These issues are highly relevant to the pulmonary circulation, which possesses the largest vascular surface area and the highest number of endothelial cells among all internal organs. Even within the lung, endothelium changes structurally and functionally along the arterial-capillary-venous axis. The richest abundance of endothelial progenitors cells is found within the lung’s capillary segment.
11.4 Endothelial Heterogeneity in the Pulmonary Circulation
Although anatomical differences in endothelium lining pulmonary arteries, capillaries, and veins were widely acknowledged in the twentieth century (Crapo et al. 1982; Haies et al. 1981; Weibel 1973), in general, lung endothelium was considered to be a functionally inert, homogeneous layer functioning primarily as a semi-permeable barrier separating blood from the underlying tissue (Gebb and Stevens 2004). Evidence that the endothelium contributes to vasoregulation and metabolism, such as the conversion of angiotensin I to angiotensin II, directed a research focus on the “non-respiratory” functions of the lung (Pfannkuch and Blumcke 1985). These studies contributed to our understanding of functional heterogeneity among lung vascular compartments; for example, lung capillaries possess extensive angiotensin converting enzyme activity (Ryan et al. 1975, 1976; Ryan and Ryan 1984a). Recognition that certain lectins discriminate between pulmonary artery and microvascular endothelial cells provided a way to test whether these cells were phenotypically different in vivo, and ultimately led to a way to purify cell phenotypes in vitro (Gebb and Stevens 2004). Lectins are plant and animal proteins that recognize sugar moieties with nominal specificity, and therefore, differential lectin binding is indicative of distinctive cell surface molecular signatures. Simultaneous infusion of labeled Griffonia simplicifolia and Helix pomatia lectins revealed a distinctive border between endothelial cell phenotypes, where pulmonary artery endothelial cells preferentially interacted with Helix pomatia and capillary endothelial cells preferentially interacted with Griffonia simplicifolia. No apparent overlap in lectin binding was noted. Recent studies seeking to resolve the transition zone found that all endothelial cells in blood vessels less than 38 μm in diameter interacted with Griffonia lectin, whereas half of the endothelial cells in blood vessels 38–60 μm in diameter interacted with Griffonia lectin, and none of the endothelial cells in blood vessels >60 μm in diameter interacted with Griffonia simplicifolia (Wu et al. 2014). These data illustrated a stark transition zone between 38 and 60 μm diameter pulmonary arterioles where a shift in endothelial cell phenotype is observed (Fig. 11.2). This transition zone resides immediately before arterioles enter the capillary plexus.
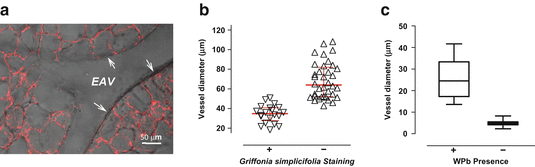
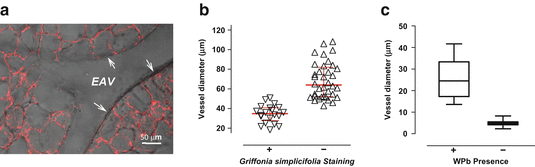
Fig. 11.2
Lung capillary endothelium is discriminated by Griffonia lectin, whereas precapillary endothelium possesses Weibel–Palade bodies. (a) The lung’s circulation was gelatin-filled and the airways were agarose-filled, thick sections were cut, and the lung slices were incubated with Griffonia lectin. Whereas endothelium lining extra-alveolar blood vessels does not interact with the lectin, capillary endothelium uniformly interacts with Griffonia simplicifolia. (b) Griffonia lectin reveals a zone, in blood vessels ranging from 38 to 60 μm, where the macrovascular endothelial cell phenotype transitions to a microvascular endothelial cell phenotype. Endothelium lining blood vessels smaller than 38 μm in diameter are uniformly Griffonia-positive, and endothelium in blood vessels greater than 60 μm in diameter is Griffonia-negative. (c) Weibel–Palade bodies are seen in precapillary endothelial cells, but are not seen in capillary endothelial cells. Adapted from Wu et al. (2014)
Weibel–Palade bodies are endothelial-specific organelles that contain many proteins important to the stimulated immune response, including P-selectin and von Willebrand factor (vWf). Interestingly, not all endothelial cells possess Weibel–Palade bodies. Fuchs and Weibel first recognized that lung capillary endothelial cells do not possess Weibel–Palade bodies, and more specifically, they found that the transition zone was in blood vessels with an approximately 20 μm internal diameter (Fuchs and Weibel 1966; Weibel 2012). The relationship between Weibel–Palade bodies and interaction with Griffonia lectin was recently compared in lung endothelium (Wu et al. 2014). In agreement with the results of Fuchs and Weibel (Fuchs and Weibel 1966; Weibel 2012), Weibel–Palade bodies were present in endothelium in small precapillary vessels, with internal diameters of approximately 18 μm. All of the endothelial cells in these blood vessels interacted with Griffonia lectin (Fig. 11.2). Thus, it appears that a macroheterogeneity among lung endothelial cell phenotypes is defined by recognition of the Griffonia lectin, although this molecular pattern does not designate the formation of Weibel–Palade bodies. These findings also resolve an important precapillary niche in lung endothelium, where a transition in phenotype occurs.
Recognition that changes in the structure of pulmonary artery and capillary endothelial cells are paralleled by distinct molecular signatures gave rise to the idea that not all endothelial cells within the pulmonary circulation are homogeneous in their function. The study of highly purified macro- and microvascular endothelial cell populations in culture has supported this view and provides insight into the rich source of endothelial progenitor cells seeded within lung capillaries.
11.5 Endothelial Heterogeneity: Insights Gained by the Study of Lung Endothelium in Culture
Mammalian cell isolation and culture has represented a breakthrough technology that is fundamental to identifying the molecular basis of cell physiology and pathophysiology. Endothelial cell cultures were not developed until the 1970s (Nachman and Jaffe 2004). Early endothelial cultures were generated from conduit-derived vessels, mostly from larger animals, because larger vessel segments were more easily accessible. What is considered normal endothelial cell identity in culture primarily arose from studies using aortic, pulmonary artery, and umbilical vein endothelial cells. In these primary cell lines, endothelial cells were shown to take up low-density lipoprotein (LDL) and to express proteins such as vWf, factor VIII, and platelet-endothelial cell adhesion molecule-1 (PECAM-1). More recently, expressions of the endothelial cell nitric oxide synthase (eNOS) and vascular endothelial cell cadherin (VE-cadherin) have been found useful in resolving endothelial identity.
Pioneering work by Ryan and colleagues paved the way for isolating capillary endothelial cells from the lungs of small animals (Habliston et al. 1979; Ryan and Ryan 1977, 1984b; Ryan et al. 1982). Using a peripheral lung cut technique and a bead retro-perfusion approach, Ryan’s group was able to obtain lung microvascular endothelial cells suitable for study in vitro. They tested the metabolic activity of these cells, including their ability to generate angiotensin II, since angiotensin-converting enzyme is largely expressed in lung capillary endothelium. These approaches have been widely adapted by investigators over the past four decades.
Comparison of lung “microvascular” (i.e., PMVECs) and “macrovascular” (PAECs) endothelial cells led to new insight in vascular biology (Stevens 2005; Gebb and Stevens 2004; King et al. 2004; Ochoa et al. 2010). Key to this insight, however, was rigorous characterization of the cell phenotype. Both PMVECs and PAECs display cobblestone morphology at confluence. Both cell types express markers characteristic of endothelium, including vWf, eNOS, VE-cadherin, and PECAM-1. However, PMVECs differ from PAECs with respect to their lectin-binding characteristics. PMVECs preferentially interact with Griffonia simplicifolia and Glycine max, whereas PAECs preferentially interact with Helix pomatia. Retention of these discriminating features is critical to the study of endothelial cell heterogeneity. It is noteworthy that most commercially available pulmonary microvascular endothelial cell lines do not report interaction with Griffonia simplicifolia in cell characterization. However, this feature of cell phenotyping is critical to resolving PMVEC behavior in culture that is reflective of its in vivo behavior. Thus, lectin-binding criteria is a critical component of endothelial cell phenotyping, especially when considering the heterogeneity of cell phenotypes.
Study of PMVECs and PAECs characterized using the aforementioned approaches has revealed that these cells differ with regard to many physiological properties, including their semi-permeable barrier function, mechanotransduction, migration, proliferation, and angiogenic capacity [reviewed in (Stevens 2005; Gebb and Stevens 2004; Ochoa and Stevens 2012; King et al. 2004; Ochoa et al. 2010)]. Rapid migration, proliferation, and angiogenic capacity is consistent with the presence of progenitor cells. Rapid PMVEC migration was noted in studies designed to evaluate mechanisms by which thrombin induces gap formation (Cioffi et al. 2002). Thrombin stimulated PMVEC gap formation within minutes of its application. However, even large micron-sized gaps were resealed within 2 h. In contrast, gap formation was slow and progressive in PAECs, and gap size was at its greatest 2 h post-thrombin challenge. Time-lapse movies revealed rapid PMVEC movement with extensive lamellipodia as cells resealed the barrier. Subsequent scratch wound assays substantiated these initial observations. From this work, it was surmised that PMVECs rapidly migrate, perhaps to sustain alveolar-capillary integrity especially following injury.
If PMVECs migrate rapidly as a cellular feature designed to protect the alveolar-capillary membrane, then they may also proliferate rapidly to repair the post-natal lung following injury. Consistent with this idea, serum-stimulated growth is greater in PMVECs than it is in PAECs, and accordingly, population doubling time during log phase growth is much shorter in the microvascular cells (King et al. 2004). In contrast to PAECs, PMVEC growth, while slowed, is not abolished in low serum conditions (0.1 %) (Solodushko and Fouty 2007). The microvascular cells do not undergo G0/G1 arrest and progress through S phase. They possess increased cdk4 and cdk2 kinase activity along with hyperphosphorylated and inactive retinoblastoma and increased cyclin D1 protein. Despite maintaining growth in low serum conditions, the establishment of a monolayer—confluence—causes G0/G1 arrest, with concomitant retinoblastoma hypophosphorylation and p27Kip1 upregulation. Thus, PMVECs possess an intrinsic growth advantage, but their growth advantage remains regulated by cell adhesion, e.g., formation of a monolayer.
11.6 Endothelial Microheterogeneity: Progenitor Cells Within a Population
The studies of Solodushko and Fouty (2007) provided mechanistic insight into what controls PMVEC progression through the cell cycle. However, their work focused on the growth characteristics of cell populations and did not seek ways to evaluate the presence, or function, of endothelial cell progenitor cells within the population. As discussed above (see Sect. 11.3), Ingram and colleagues (2004) utilized single cell cloning approaches to establish growth hierarchies within endothelial cell populations, and thereby developed functional assays to identify endothelial progenitors. Alvarez and colleagues (2008) adapted this approach for evaluation of PMVEC and PAEC progenitor growth characteristics.
Using PMVEC and PAEC populations, characterized as described (see Sect. 11.5), Alvarez and colleagues (2008) subjected cells to the clonogenic assay and established a hierarchy of growth potentials among single cells. Whereas the hierarchy of PAEC growth potentials was similar to previous descriptions in HUVECs, with <10 % of cells displaying a high proliferative potential, nearly 50 % of single PMVECs were highly replication competent (Fig. 11.3). Similar results were obtained in endothelial cells isolated from mice (Schniedermann et al. 2010). These data suggest that endothelial cells isolated from lung capillaries possess an intrinsically high proliferative potential with the capacity to self-renew, distinguishing characteristics of progenitor cells.
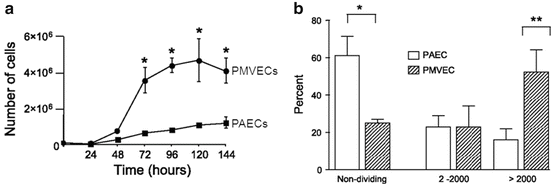
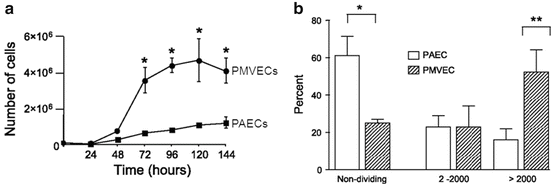
Fig. 11.3
Pulmonary microvascular endothelial cell populations contain a high percentage of progenitor cells that contribute to their rapid proliferation. (a) PMVEC populations proliferate rapidly when compared to PAEC populations. Cells were seeded at 105 cells in 35 mm dishes, and serum (10 %)-stimulated growth evaluated over 6 days. (b) Single cell clonogenic assay reveals a high percentage of replication competent PMVECs; as many at 50 % of PMVECs display a high proliferative capacity. Adapted from Alvarez et al. (2008)
PMVECs display a restrictive barrier property, limiting trans-endothelial water, solute and protein flux. However, it is generally believed that cell adhesion limits cell proliferation. High proliferative potential cells isolated from PMVECs were tested to determine whether they establish a restrictive barrier (Alvarez et al. 2008). Similar to the parent cell population, high proliferative potential cells generated a restrictive barrier. These cells also retained expression of usual endothelial “marker” proteins, such as VE-cadherin, vWf, PECAM-1, eNOS, and N-cadherin. They retained an ability to interact with Griffonia simplicifolia and did not readily interact with Helix pomatia, similar to the parent cells. High proliferative potential cells expressed proteins characteristic of circulating endothelial progenitor cells, including vascular endothelial cell growth factor receptor-2 and CD105, but they did not express CD133 or CD45. Thus, high proliferative potential cells were selected from a population of lung microvascular endothelial cells, PMVECs, and while these individual cells grew rapidly and fulfilled the criterion of progenitor cells, they maintained their “microvascular” identity.
High proliferative potential endothelial cells were tested to determine whether they displayed evidence of replicative senescence (Alvarez et al. 2008). Short telomeres are associated with replicative senescence. Telomeres were fluorescently labeled, and their length evaluated against control cell populations. PAECs displayed the shortest telomere lengths and high proliferative potential PMVECs possessed the longest telomere lengths, consistent with their rapid proliferative capacity. Growth in soft agar matrix was measured to evaluate possible cell transformation. Whereas breast cancer cells grew into large, anchorage-independent clumps, growth was suppressed in PAECs, PMVECs, and high proliferative potential PMVECs, suggesting high proliferative potential cells displayed regulated cell cycle progression. Consistent with this idea, as high proliferative potential PMVECs grew to confluence in a serum (10 %)-stimulated growth curve, the percentage of S phase cells decreased substantially (e.g., cells came out of the cell cycle), similar to both PAECs and PMVECs. Both PMVECs and high proliferative potential PMVECs were rapidly neo-angiogenic as well, when compared to PAECs. However, selection of high proliferative potential cells did not enrich for a cell population with even greater angiogenic benefit, as both the parent PMVEC population and the high proliferative potential PMVECs generated similar numbers of blood vessels.
Studies seeking to resolve a molecular basis for rapid endothelial growth identified increased expression of nucleosome assembly protein 1 in PMVECs and in high proliferative potential PMVECs (Clark et al. 2008). Nucleosome assembly protein 1 is an epigenetic, pro-proliferative factor that is conserved from yeast to mammals. This protein was detected in a comparative mRNA profiling transcript analysis in PAECs and PMVECs. In the initial screen, nucleosome assembly protein 1 was highly expressed in PMVECs, even at confluence. Nucleosome assembly protein 1 downregulation decreased the proliferative and neo-angiogenic capacity of PMVECs, and its overexpression increased the proliferative and neo-angiogenic capacity of PAECs. In both experimental cases, however, changing nucleosome assembly protein 1 expression did not impact the expression of endothelial cell markers, including PECAM-1, VE-cadherin, and vWf. It also had no effect on lectin-binding criteria; PAECs recognized Helix pomatia even following nucleosome assembly protein 1 overexpression, and PMVECs recognized Griffonia simplicifolia even following nucleosome assembly protein 1 silencing. These findings implicate nucleosome assembly protein 1 as an epigenetic determinant of endothelial growth, but not in either endothelial or “microvascular” versus “macrovascular” phenotype specifications. Studies have not yet been completed to evaluate how nucleosome assembly protein 1 impacts the clonogenic potential, or the hierarchy of growth potentials, among single endothelial cells.
Endothelial cells that display rapid growth characteristics, such as PMVECs, can easily be identified in culture systems by the color of their medium at post-confluence. Mammalian cell culture medium contains phenol red, which is red at normal pH, light red-to-pink with alkalotic pH, and yellow with acidotic pH. The medium of post-confluent PMVECs becomes increasingly yellow, suggesting development of a lactic acidosis. This observation prompted investigation into the means by which PMVECs sustain bioenergetic demands during rapid growth (Parra-Bonilla et al. 2010, 2013). Microarray, RT-PCR, and western blot analysis revealed increased expression of glycolytic enzymes in PMVECs when compared with PAECs. Moreover, as cells grew to confluence in a standard serum-stimulated growth curve, PMVECs consumed glucose from the medium, generated lactate, and produced acidosis. Oxygen consumption was lower in PMVECs than it was in PAECs, and yet total ATP concentrations were higher in the microvascular cells. These data suggest that PMVECs, and likely high proliferative potential PMVECs, rely on glucose fermentation to sustain their rapid proliferation.
To test this idea more directly, glucose was restricted from the PMVEC medium during growth (Fig. 11.4). Glucose restriction produced a dose-dependent decrease in cell growth and attenuated the lactate accumulation and acidosis. Moreover, galactose substitution for glucose abolished the lactic acidosis and greatly reduced cell proliferation, while reducing PMVEC ATP concentrations. Interestingly, supplying extracellular lactate to the galactose-treated PMVECs rescued ATP concentrations. Glucose consumption and the reliance on glycolysis to sustain ATP concentrations and cell proliferation are consistent with the use of aerobic glycolysis to sustain the bioenergetic demands of PMVEC growth.
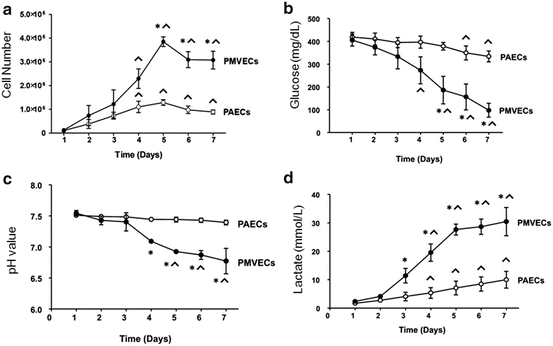
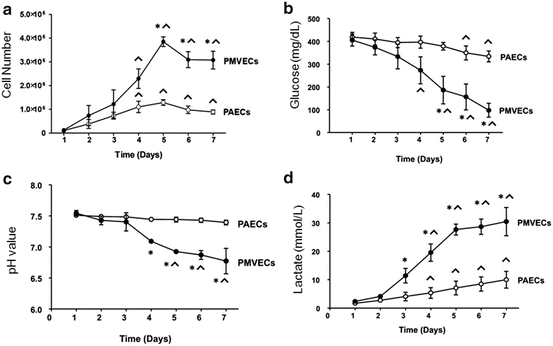
Fig. 11.4
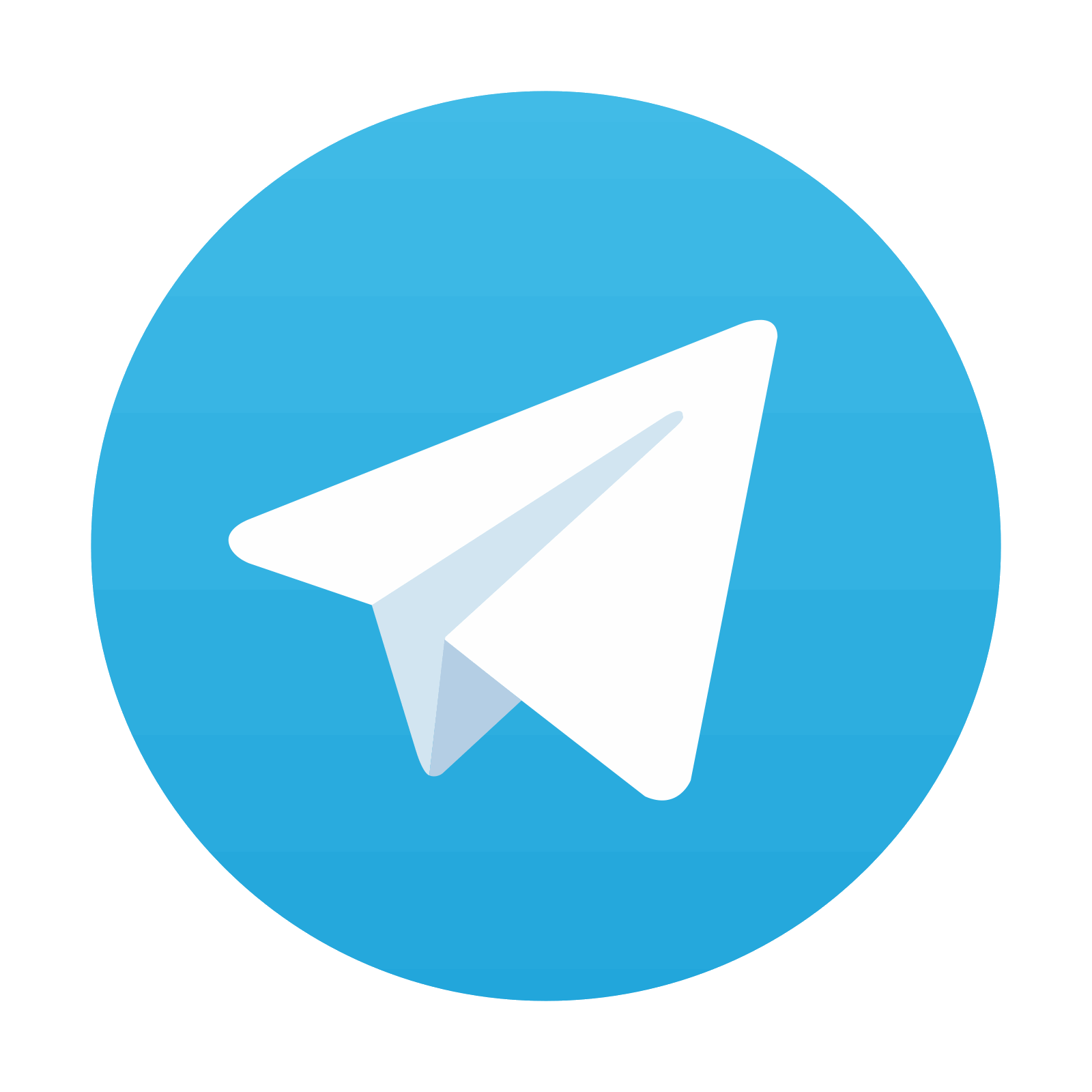
Pulmonary microvascular endothelial cells utilize aerobic glycolysis to sustain their bioenergetic demands during proliferation. As PMVECs undergo rapid proliferation (a), they consume glucose from the media (b), produce an acidosis (c), and generate lactate (d). These features, including glucose consumption and production of lactic acidosis in the presence of sufficient oxygen, are characteristic of aerobic glycolysis. Adapted from Parra-Bonilla and coworkers (2010)
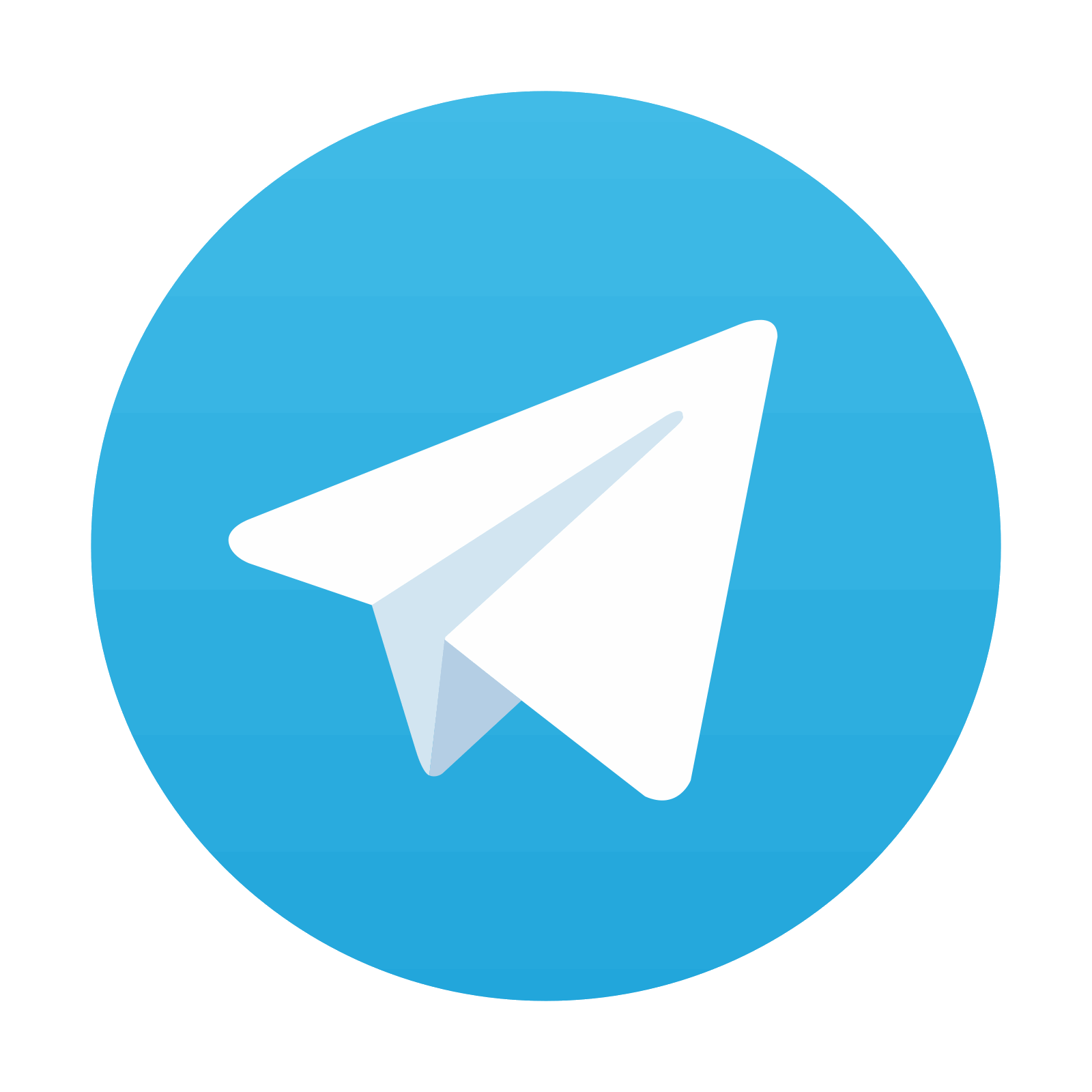
Stay updated, free articles. Join our Telegram channel
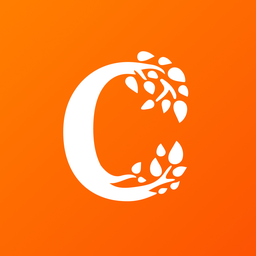
Full access? Get Clinical Tree
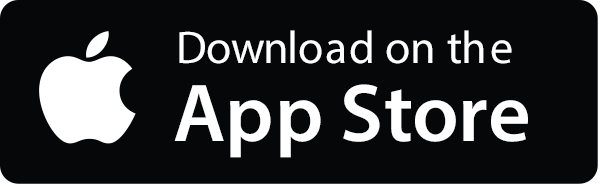
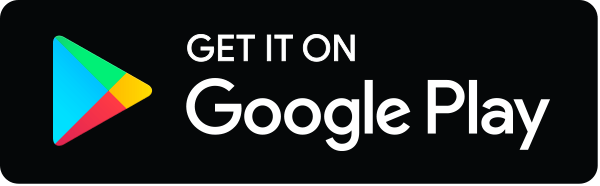
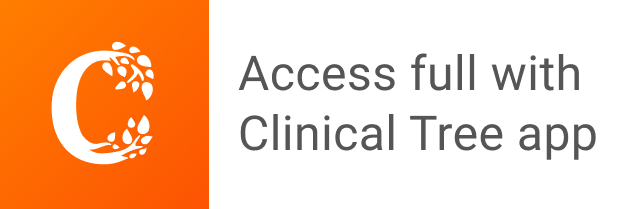