Fig. 8.1
Fluorescence microscopy imaging techniques used for intravital microscopy. a Confocal microscopy. (Top) In confocal microscopy, excitation and emission occur in a relative large volume around the focal plane (yellow triangles). The off-focus emissions are eliminated through a pinhole. (Bottom) In 1P microscopy, a fluorophore absorbs a single photon with a wavelength in the UV–visible range of the spectrum (purple arrow). After a vibrational relaxation (black arrow), a photon with a slightly shorter wavelength is emitted (blue arrow). Confocal microscopy enables imaging at a maximal depth to 80–100 µm. b Two-photon microscopy. (Top) Emission and excitation occur only at the focal plane in a restricted volume (yellow spot), and for this reason a pinhole is not required. (Bottom) In this process a fluorophore absorbs almost simultaneously two photons that have half of the energy (twice the wavelength) (red arrow) required for its excitation with a single photon. Two-photon excitations typically require IR light (from 700 to 1500 nm). Two-photon microscopy enables imaging at a maximal depth of 300–500 µm
Two – photon microscopy

Fig. 8.2
Examples of fluorescence intravital microscopy applications to study lung injury. Characteristics of acute lung injury are observed after intratracheal instillation with MRSA (Methicillin-resistant Staphylococcus aureus −5 × 107 cfu). a Vascular permeability, observed by leakage into the alveolar space of i.v. Cascade Blue dextran. b Neutrophil elastase activity is monitored by the cleavage of a far red fluorescent substrate (NE680 FAST, Perkin Elmer), shown in pink in MRP8-Cre × mTmG lungs (GFP: neutrophils, tdTomato: ubiquitous). c Neutrophil recruitment is observed during the course of infection in MRP8-Cre × mTmG lungs at 3 and 5 h after infection. White bars indicate 30 μm
Lung Fluorescence Imaging Preparations
Technological advances in microscopy allow the acquisition of high-resolution images of the subpleural layers of the lung to analyze cellular and subcellular processes. Lung fluorescence imaging preparations can take three main forms: explanted tissues, live lungs slices placed under the flow of suitable medium, and intravital imaging where the lung is maintained in its natural microenvironment.
Live Lung Slices (LLS)
Description of LLS Preparation
The live lung slice method consists of partitioning the lung in thick slices, which are then carefully maintained to assure tissue viability. To do so, after the exsanguination of the animal, a cannula is placed intratracheally and lungs are inflated with 1.5–2 % liquid low melting agarose to maintain lung structure. The lungs are extracted and agarose is solidified in cold physiological medium. With the use of a micro-slicer or a vibratome, 150–300 μm thick serial slices are obtained. The vibration offers the possibility of cutting living tissue with a minimal injury. The slices are maintained in cell culture media at 37 °C and 5 % CO2. Lung slices are normally used in the first 8 h although they have been used up to 2–3 days after sampling [9]. The lung slices have normal cell activity allowing the study of cell interactions and at the same time the structure of the alveoli is maintained to allow the 3D imaging of cell movements. For complete tissue preparation methods review, please see Thornton et al. [10].
Advantages and Disadvantages of LLS
LLS preparations have several advantages in comparison to fixed tissue imaging. First, fixed tissue samples are usually much thinner than live lung slices. The increased thickness of the live slices allows the study of three-dimensional cell movements that would be lost in thinner preparations or cellular monolayers. Second, and more importantly, unlike the static fixed images, LLS allow the study of time-dependent processes ranging from fast cell–cell interactions to the study of complex and slow pathogenic immune responses in the lung. Third, the LLS technique is a useful tool to study cellular interactions deep in the lung where intravital imaging or the isolated, perfused lung preparation cannot reach due to the excessive thickness. Because only a section of lung is studied with LLS preparations, it has important limitations such as the loss of blood and lymph circulation and the lack of neural input. These considerations are very important in studies requiring cell egress from other organs or other parts of the lung. Leukocyte activation induced by the isolation procedure may also be a concern.
Application of LLS in Lung Injury Studies
Cell death analysis
Ca 2 + measurement
Tissue / cell identification
Transgenic mouse studies
Isolated, Perfused Lung Method (IPL)
Description of the IPL Method
In the isolated, perfused lung preparation, the left pulmonary artery is cannulated and perfused with autologous blood or a physiologic salt solution allowing passive drainage through the left ventricle. The lungs are ventilated with normal air or humidified gas containing different concentrations of oxygen and positive end-expiratory pressure is maintained. Using a perfusion pump, experimental agents can be added to the perfusate and introduced in the lung circulation. The lungs are suspended from a force displacement transducer to measure lung weight changes, while the whole system is maintained in a closed chamber with controlled humidity and temperature.
Advantages and Disadvantages of IPL
The main advantage of IPL technique over the live intravital approach is the unmatched stability of the sample. This important feature has permitted the use of directed microinjections of fluorescent dyes into endothelial or epithelial cell layers in the lungs [16]. Perfused whole lung preparations are a better approach to study lung physiology than LLS preparations. IPL preparations do not have the injured cut surfaces at the top and bottom of the preparation, which may induce undesirable effects when studying organ functionality. Vascular leakage is also better studied in isolated lungs than in LLS [17]. A clear disadvantage of isolated lung preparations is that it lacks the effects of the normal (or induced) physiological changes that occur systemically. This effect diminishes the possibility of leukocyte chemoattraction to the lungs from the bone marrow and circulation, which is an important limitation in inflammation-related studies. A possible leukocyte activation effect induced by the pump-assisted circulation used in the preparation of IPL is another disadvantage when compared with lung intravital microscopy [18].
Application of IPL to Lung Injury Models
Tissue / cell identification
Leukocyte trafficking
ROS production
NO production
Lung Intravital Microscopy Methods (IVM)
Description of the Lung IVM Method
To observe the lung in a more physiological manner, it should be imaged in vivo. As discussed earlier, challenges faced with lung imaging compared to other organs are its intrinsic motion and the maintenance of breathing after opening the thorax. Different approaches have been developed to address these issues including (a) maintaining mechanical ventilation or reestablishment of spontaneous breathing, (b) the structure and composition of the window in contact with the lung, and (c) the management of cardiorespiratory motion.
Ventilation and closed thorax imaging
Thoracic windows specificities
Control of lung motion
Wagner et al. [8] addressed in an efficient way the obstacle of cardiorespiratory movement in live animal imaging using a thoracic window with built-in suction, providing enough stabilization for real-time microscopy. A similar technique, adapted from Wagner’s thoracic window coupling suction to gently immobilize the lung on a glass coverslip has been recently adopted and miniaturized for mice by Looney et al. [24]. Different mouse windows were developed by groups in Germany [26], Japan [29] and USA [24, 27, 31]. The application of these lung intravital microscopy methods to mice has allowed access to the tools available with transgenic animals.
Advantages and Disadvantages of Intravital Microscopy
Advantages
Disadvantages
Applications of IVM for Lung Injury Models
With these advanced fluorescence microscopy methods, it is possible to generate high-resolution images of several z-stack positions to generate 3D reconstitutions. The methods described also allow for imaging over several hours to generate time-lapsed data. It is then possible to analyze, localize, and quantify different parameters in four dimensions (3D plus time) such as cell velocity, colocalization, shape, volume, number, intensity, and color. Fluorescence is defined as the emission of light from a fluorescent probe after its excitation by an external light source of defined wavelength. The visualization of tissues, cells or proteins can be achieved by tagging molecules with specific fluorophores which can be selectively excited and specifically visualized. Here, we describe some useful applications for lung injury models.
Lung morphology and structural changes
Lung edema and vascular leakage
Leukocyte recruitment
Table 8.1
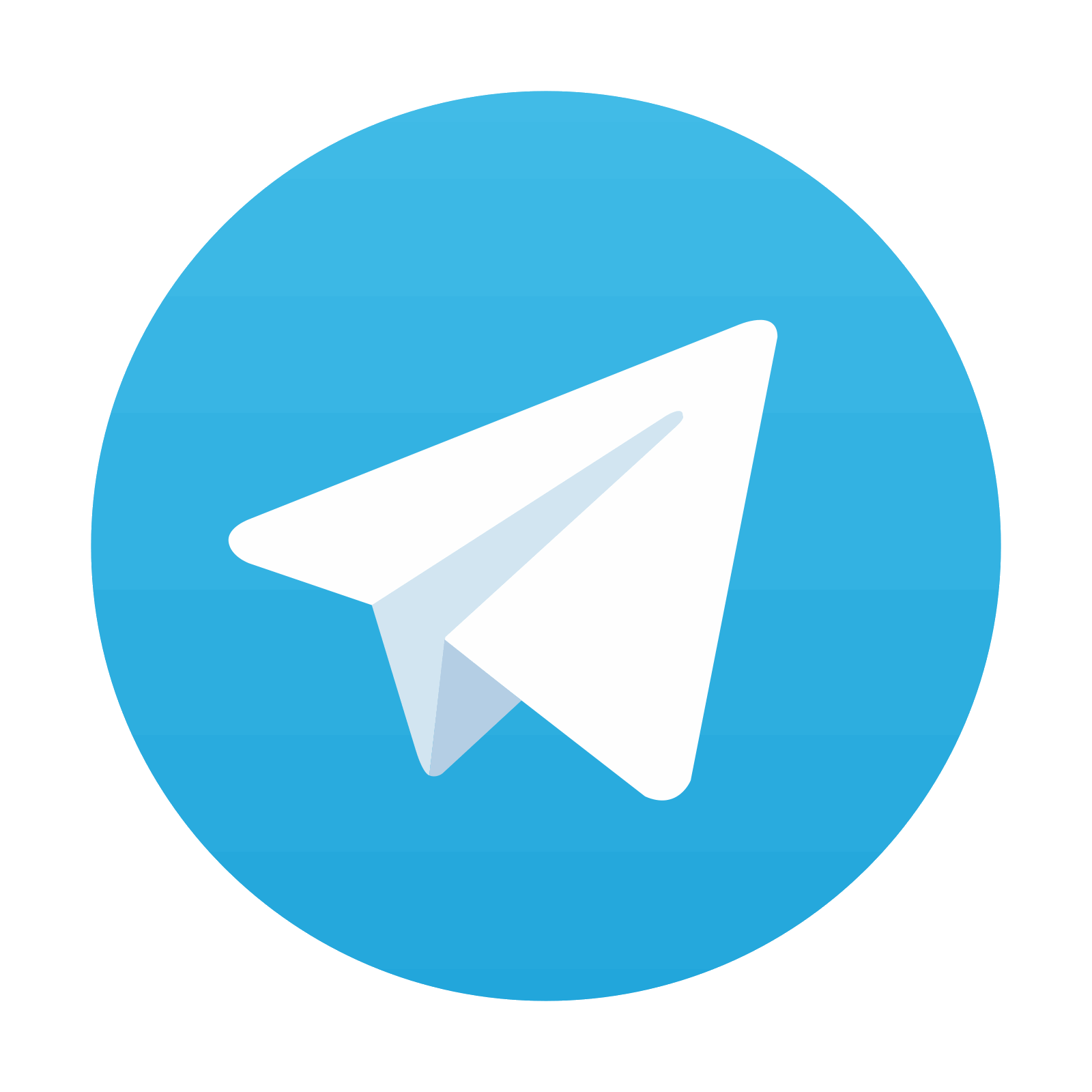
Fluorescent transgenic mice commonly used for lung injury models
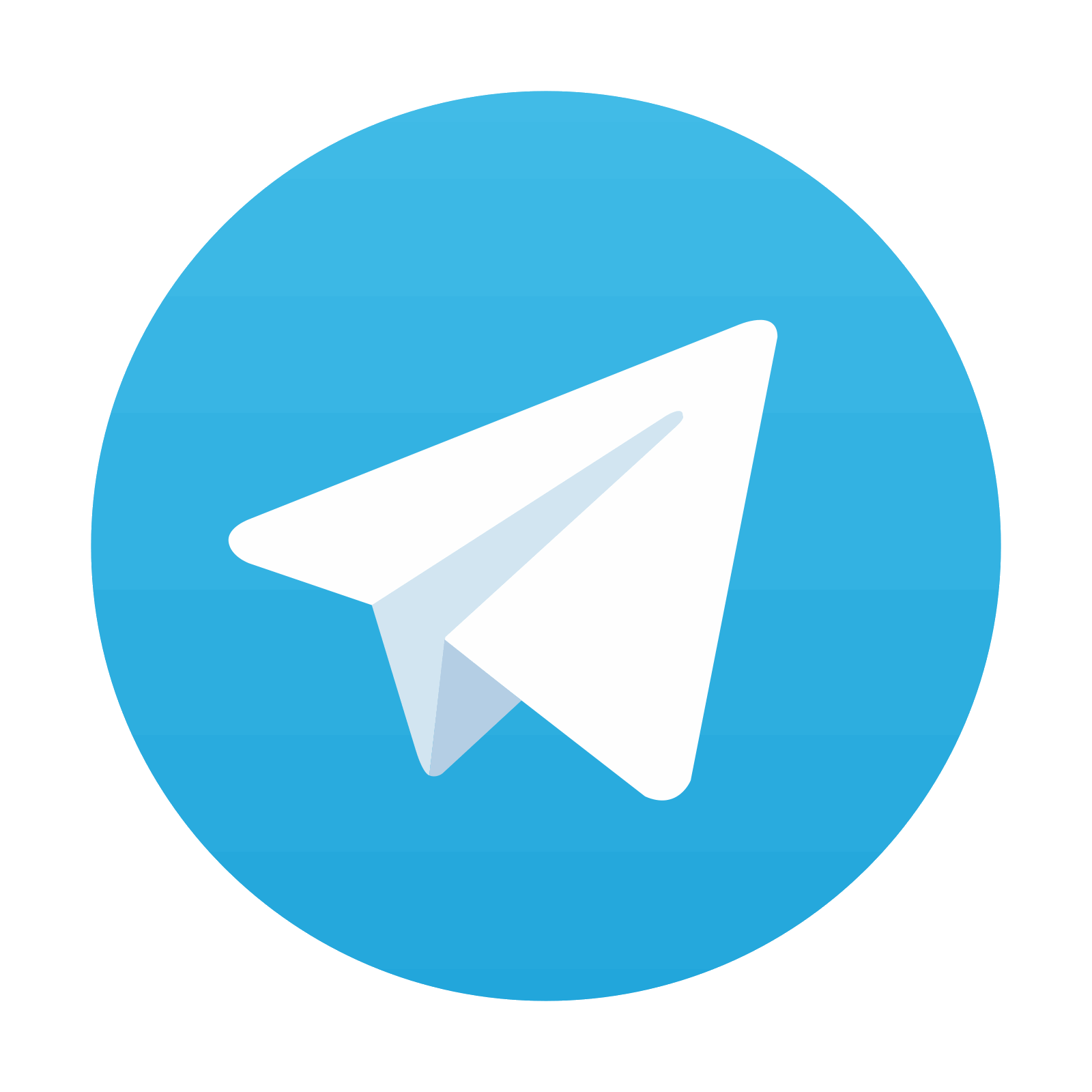
Stay updated, free articles. Join our Telegram channel
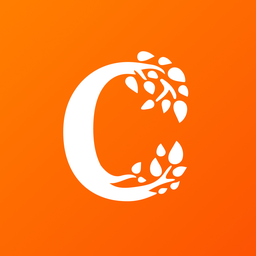
Full access? Get Clinical Tree
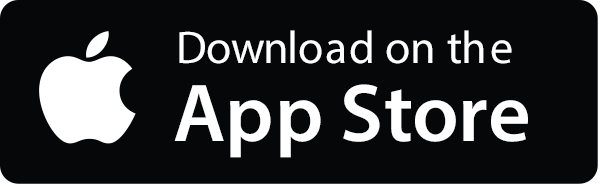
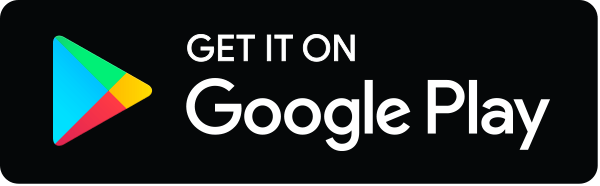
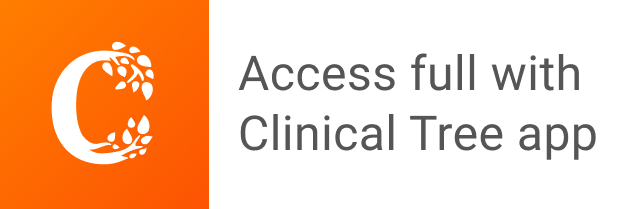