Background
Although diabetes mellitus is well known to result in systolic and diastolic left ventricular (LV) dysfunction at the subclinical level, even when it is not accompanied by hypertension and coronary artery disease, this situation has not been sufficiently investigated in prediabetes, which is the precursor of diabetes. The aims of the present study were to investigate LV systolic and diastolic function in normotensive and low-risk prediabetic and diabetic subjects for coronary disease using sensitive tissue Doppler echocardiographic parameters, to investigate early possible negative effects of glucose metabolism impairment on LV longitudinal function.
Methods
Two hundred subjects (92 with prediabetes, 48 with type 2 diabetes, and 60 age-matched healthy volunteers) were studied by conventional, tissue Doppler, and strain and strain rate echocardiography. All study subjects were normotensive, and coronary artery disease was excluded. Forty-eight patients had isolated fasting glucose impairment, and 44 patients had combined fasting glucose and glucose tolerance impairment. Longitudinal peak systolic strain and the peak systolic and diastolic strain rates of six walls in the apical four-chamber, long-axis, and two-chamber views were evaluated.
Results
Clinical and standard echocardiographic characteristics were comparable among all groups. Mean systolic ( P = .01) and diastolic ( P = .02) tissue velocities, mean strain ( P = .004), and mean systolic ( P = .002) and diastolic ( P = .001) strain rates were significantly lower in the diabetic groups than in control subjects. There were no difference between patients with isolated fasting glucose impairment and controls for tissue Doppler parameters, but mean early diastolic tissue velocity and mean strain and strain rates were statistically lower in patients with combined fasting glucose and glucose tolerance impairment compared with controls ( P < .05).
Conclusions
LV longitudinal systolic and diastolic function was impaired in both normotensive diabetic and prediabetic patients.
Diabetes mellitus (DM) is associated with significantly higher heart failure risk, and its prevalence is growing rapidly. Increased ratios of heart failure in diabetic patients persist despite corrections for age, hypertension, obesity, hypercholesterolemia, and coronary artery disease (CAD). It is believed that diastolic dysfunction is an early complication in type 2 DM, and it has been suggested to be the first stage in the development of diabetic cardiomyopathy. Diabetic cardiomyopathy is characterized by defects in both diastolic and systolic function, even in the absence of CAD, hypertension, and left ventricular (LV) hypertrophy. Thus, it is defined as LV dysfunction that occurs independently of CAD and hypertension. Diabetic cardiomyopathy is progressive and involves a progression from the normal heart to preclinical LV diastolic and systolic dysfunction (diagnosed only using advanced imaging techniques), followed by clear echocardiographic evidence of LV dysfunction (still clinically silent) and eventual symptomatic heart failure. Several authors have shown disturbances in subtle indices of systolic and diastolic function, including tissue Doppler, strain, and strain rate, in patients with type 2 DM.
Prediabetes is a metabolic intermediate state between normal glucose tolerance and overt type 2 DM. Impaired fasting glucose (IFG) and impaired glucose tolerance (IGT) are prediabetic states. According to the American Diabetes Association, patients with isolated IFG (i-IFG) have fasting plasma glucose (FPG) levels between ≥100 and <126 mg/dL and 2-hour plasma glucose levels (measured by an oral glucose tolerance test [OGTT]) <140 mg/dL. Individuals with IGT have FPG concentrations <100 mg/dL and 2-hour plasma glucose concentrations, measured by 75-g OGTT, ranging between ≥140 and <200 mg/dL. IFG and IGT often coexist. Prediabetes is considered a substantial risk factor for diabetes and is associated with microvascular complications and cardiovascular disease in a way similar to DM. Although the relationship between DM and preclinical LV dysfunction has been well described and documented in numerous studies, data regarding LV dysfunction in patients with prediabetes are incomplete, and no studies thus far have evaluated the relationship between subgroups of prediabetes (including IFG and/or IGT) and subtle changes in LV myocardial function.
Therefore, the main aim of the present study was to investigate the possible effect of glucose metabolism impairment on LV systolic and diastolic function from the initial stages to diabetes. Because cardiac involvement in diabetes seems to represent a continuum of preclinical stages, we particularly sought an association between glucose metabolism status and LV tissue Doppler parameters. To investigate the early alterations in LV function in prediabetic and diabetic patients without systemic hypertension and without CAD, echocardiographic strain and strain rate techniques were used.
Methods
Subjects
All patients with diabetes and prediabetes prospectively enrolled between November 2010 and February 2011 were admitted to diabetes and cardiology outpatient clinics. Categories of abnormal glucose metabolism were determined according to the 2003 guidelines of the American Diabetes Association. Prediabetic patients were classified into two groups on the basis of OGTT results: those with i-IFG and those with combined IFG and IGT (IFG+IGT).
The study population consisted of four groups: 48 patients with i-IFG, 44 patients with IFG+IGT, 48 patients with type 2 DM, and 60 subjects as age-matched and gender-matched controls.
CAD was excluded by negative results on dobutamine stress echocardiography, treadmill exercise tests, and coronary angiography.
At the beginning of the study, all prediabetic and diabetic patients were subjected to both dobutamine stress echocardiography and treadmill exercise tests. Coronary angiography was carried out when the results of one or both of the tests were positive and/or when patients described angina pectoris. As a result, 100 patients (30 from the DM group, 36 from the i-IFG group, and 34 from the IFG+IGT group) had negative result on both dobutamine stress echocardiography and treadmill exercise tests, and 40 patients (18 from the DM group, 12 from the i-IFG group, and 10 from the IFG+IGT group) had normal results on coronary angiography. In addition, on 24-hour Holter monitoring, no subjects showed any signs of myocardial ischemia.
On the basis of repeated blood pressure measurements, all patients and control subjects had normal arterial blood pressure (≤130/85 mm Hg or less) and were receiving no blood pressure–lowering medications. All were in sinus rhythm, with normal results on 12-lead electrocardiography, without signs of hypertrophy, bundle branch block, or pathologic Q waves. All had normal transthoracic echocardiographic results. Control subjects had normal results on physical examination; had no chronic cardiovascular illnesses, including hypertension and diabetes; had no symptoms or histories suggestive of cardiovascular disease; had normal results on dobutamine stress echocardiography and treadmill exercise tests; and were considered to have a low probability of coronary disease on clinical grounds.
Patients were excluded if they had diabetic complications such as retinopathy, clinical neuropathy, or macroalbuminuria; positive results for myocardial ischemia by dobutamine stress echocardiography or treadmill exercise testing; significant CAD (quantitative coronary angiographic stenosis diameter > 40%); regional wall motion abnormalities or impaired ejection fractions (<55%) by resting echocardiography; poor-quality echocardiographic imaging; valvular or congenital heart disease; previous surgical or percutaneous revascularization; or endocrine and systemic diseases other than DM. All other eligible patients were studied.
The study was conducted in accordance with the Helsinki II Declaration and approved by the local ethics committee. All participants provided written informed consent before entering the study.
Blood for biochemical analysis was obtained from fasting venous samples. Glucose, glycosylated hemoglobin (HbA 1c ), total cholesterol, high-density lipoprotein, and triglycerides were determined using standard laboratory techniques. Standard 75-g OGTTs were performed after an overnight fasting period of 10 hours.
Classification of IFG and IGT categories was made on the basis of American Diabetes Association criteria as follows: (1) for i-IFG, FPG of 100 to 125 mg/dL (5.6–6.9 mmol/L) and 2-hour postprandial plasma glucose <140 mg/dL (17.8 mmol/L); (2) for IFG+IGT, FPG of 100 to 125 mg/dL (5.6–6.9 mmol/L) and 2-hour postprandial plasma glucose of 140 to 199 mg/dL (7.8–11 mmol/L); and (3) for diabetes, FPG ≥ 126 mg/dL (7 mmol/L) and/or 2-hour postprandial plasma glucose ≥ 200 mg/dL (11.1 mmol/L).
Echocardiography
All subjects were examined in the left lateral decubitus position, using a System Five machine (GE Vingmed Ultrasound AS, Horten, Norway) with a 2.5-MHz multifrequency transducer. Electrocardiograms were recorded simultaneously. Echocardiographic data were taken during passively held end-expiration, which contained a minimum of three consecutive beats and were stored digitally. All measurements were analyzed by an experienced echocardiographer without knowledge of the clinical data.
Standard echocardiographic assessment included M-mode, cross-sectional, and Doppler blood flow measurements. LV volumes were calculated using the modified Simpson’s biplane method of disks, and LV mass was calculated using the Devereux-modified American Society of Echocardiography cube formula. LV volumes and LV mass were indexed for body surface area to obtain the LV end-diastolic volume index, LV end-systolic volume index, and LV mass index. LV ejection fraction was estimated using a modified Simpson’s biplane method. LV fractional shortening was calculated using a standard formula. Pulsed-wave Doppler of transmitral flow was carried out in the apical four-chamber view. The sample volume was placed at the tips of the mitral leaflets, and peak early diastolic mitral flow velocity (E), peak late diastolic mitral flow velocity (A), and deceleration time of early diastolic mitral flow were recorded. The E/A ratio was calculated.
Doppler tissue imaging was obtained from four-chamber and two-chamber apical views and the apical long-axis view during end-expiration apnea. Color tissue Doppler was superimposed on two-dimensional images. The width of the image sector and the depth of the imaging were adjusted to achieve a color Doppler frame scanning rate > 140 frames/sec. Pulse repetition frequency was set to the lowest possible value without aliasing. If found, increasing the pulse repetition frequency (typically between 1 and 2 kHz) eliminated the aliasing. The sampling window was positioned as parallel as possible to myocardial segment of interest to ensure the optimal angle of imaging. Myocardial tissue velocity, strain rate, and strain analysis were performed offline using customized computer software (EchoPAC version 6.3; GE Vingmed Ultrasound AS). Cine loops containing three consecutive cardiac cycles were analyzed, and all measurements were taken as the mean of the three consecutive cycles.
Myocardial tissue velocities were measured in the basal segments of the lateral, septal, anterior, inferior, posterior, and anteroseptal LV walls. Peak systolic velocity during ejection and the peak diastolic velocities during early filling from the waveforms were obtained. The average of these four basal velocities was used to calculate mean basal peak systolic velocity (Sm) and mean basal peak early diastolic velocity (Em). As a noninvasive estimate of LV end-diastolic pressure, the ratio of mitral inflow early diastolic velocity (E) to mean basal peak early diastolic velocity (Em) was determined.
Strain and strain rate curves were extracted from color tissue Doppler images by standard software (EchoPAC). Strain and strain rate were derived from strain and strain rate curves obtained by placing a sample volume (9.2 mm) in the basal and mid segments from the three apical views. The sample volume was located in the central part of each segment and tracked manually throughout the cardiac cycle to improve the accuracy of the technique. The peak systolic strain rate was assessed as an average of the values obtained from three consecutive heart cycles. The peak systolic strain rate in each segment was assessed as the lowest value between the R wave on the electrocardiogram and closure of the aortic valve, which was defined by a curved anatomic M-mode recording placed through the aortic valve leaflets in the apical long-axis view. We excluded data if we were unable to obtain a smooth strain curve or the angle between the scan line and wall was >20°. A total of 12 segments were assessed (mid and basal segments of the lateral, septal, anterior, inferior, posterior, and anteroseptal LV walls) in all study subjects, and mean peak systolic strain was calculated by dividing the sum of each longitudinal peak strain values to the number of used segments as global longitudinal LV systolic indices. In the same way, mean peak systolic strain rate and mean peak early diastolic strain rate, referred to as LV global systolic and diastolic indices, respectively, were computed. This methodology for obtaining global LV functional parameters is supported by a previous study, which demonstrated a strong association between the strain rate at the basal septum and peak elastance, which was the gold standard of global LV contractility. In another study, mean myocardial tissue Doppler velocities obtained from the basal segments of the left ventricle seemed to be the most feasible and accurate indices for routine assessment of diabetic myocardial disease.
To define the intraobserver and interobserver variability, the strain and strain rate measurements were repeated for 15 randomly selected patients after 4 weeks by the same observer on the same echocardiographic images and by a second independent observer. Intraobserver variability for the measurement of the peak systolic strain, peak systolic strain rate, and peak early diastolic strain rate was 4.4 ± 3.6%, 5.4 ± 3.7%, and 5.6 ± 3.3%, respectively. Interobserver variability for these same measurements was 4.9 ± 3.7%, 6.2 ± 3.1%, and 6.4 ± 2.8%, respectively ( Table 1 ).
Variable | Intraobserver variability (%) | Interobserver variability (%) |
---|---|---|
Peak systolic strain | 4.4 ± 3.6 | 4.9 ± 3.7 |
Peak systolic strain rate | 5.4 ± 3.7 | 6.2 ± 3.1 |
Peak early diastolic strain rate | 5.6 ± 3.3 | 6.4 ± 2.8 |
Statistical Analyses
Statistical analyses were performed using SPSS version 15.0 (SPSS, Inc., Chicago, IL). Data are presented as mean ± SD, except for diabetes duration, which is presented as the geometric mean. Frequencies are expressed as percentages. To compare the variables derived from the clinical characteristics and standard echocardiographic examinations between the IFG, IGT, IFG+IGT, and normal groups, one-way analysis of variance and post hoc Bonferroni tests were used. Because body mass index (BMI) was significantly higher in the IFG+IGT and DM groups than in the control group, analysis of covariance was used to adjust for BMI to compare LV tissue Doppler parameters among groups. Student’s independent-samples t tests were used to compare differences between two groups. Categorical variables were compared using Fischer’s exact tests or χ 2 tests as appropriate. Pearson’s correlation coefficient ( r ) was used to estimate correlations between individual parameters. Two-tailed P values < .05 were considered significant.
Results
The clinical characteristics of the prediabetic, diabetic, and control subjects are summarized in Table 2 . There were no statistical differences in gender, age, heart rate, systolic, diastolic, and mean blood pressure, and lipid profile among disease and control groups. The median DM diagnosis duration was 5 years (range, 2–12 years). BMI was significantly higher in diabetic ( P = .002) and IFG+IGT ( P = .042) patients than in the control group but did not differ between i-IFG and control groups. FPG was significantly higher in the diabetic group than in other groups ( P < .001). The i-IFG and IFG+IGT groups also had significantly higher FPG than the control group ( P < .001). There were no statistical differences in FPG between the i-IFG and IFG+IGT groups. HbA 1c was significantly higher in the diabetic group than the i-IFG and IFG+IGT groups ( P < .001).
Variable | Controls ( n = 60) | i-IFG ( n = 48) | IFG+IGT ( n = 44) | DM ( n = 48) | P |
---|---|---|---|---|---|
Age (y) | 56 ± 11 | 56 ± 10 | 57 ± 11 | 56 ± 11 | NS |
Men | 53% | 54% | 57% | 58% | NS |
BMI (kg/m 2 ) | 27.2 ± 3.8 | 28.3 ± 3.7 | 29.4 ± 3.8 | 30.2 ± 3.7 | .001 ∗ |
Heart rate (beats/min) | 72 ± 9 | 74 ± 9 | 73 ± 9 | 72 ± 8 | NS |
Systolic BP (mm Hg) | 122 ± 9 | 124 ± 12 | 124 ± 11 | 126 ± 12 | NS |
Diastolic BP (mm Hg) | 72 ± 8 | 73 ± 6 | 73 ± 7 | 74 ± 7 | NS |
Duration of diabetes (y) | — | — | — | 5 (2–12) | — |
FPG (mg/dL) | 84 ± 8 | 108 ± 7 | 110 ± 8 | 144 ± 31 | <.001 † |
HbA 1c (%) | — | 5.2 ± 0.5 | 5.7 ± 0.6 | 7.8 ± 1.6 | <.001 ‡ |
Cholesterol (mg/dL) | 190 ± 46 | 194 ± 39 | 202 ± 42 | 188 ± 42 | NS |
LDL cholesterol (mg/dL) | 118 ± 30 | 122 ± 32 | 130 ± 32 | 116 ± 30 | NS |
HDL cholesterol (mg/dL) | 54 ± 12 | 52 ± 11 | 50 ± 12 | 48 ± 12 | NS |
Triglycerides (mg/dL) | 165 ± 61 | 168 ± 64 | 172 ± 65 | 186 ± 62 | NS |
∗ Significant difference between DM and control groups and between IFG+IGT and control groups.
† Significant difference compared with controls and between DM and i-IFG groups and between DM and IFG+IGT groups.
‡ Significant difference between DM and i-IFG groups and between DM and IFG+IGT groups.
Standard echocardiographic characteristics of the study groups are given in Table 3 . All measurements were within normal limits. There were no differences between groups in left atrial diameter, LV systolic or diastolic dimensions, LV ejection fraction, LV fractional shortening, and LV mass index. Mitral inflow velocities (E, A, E/A, and deceleration time of early diastolic mitral flow) were also similar between the groups.
Variable | Controls ( n = 60) | i-IFG ( n = 48) | IFG+IGT ( n = 44) | DM ( n = 48) | P |
---|---|---|---|---|---|
LAD (mm) | 36 ± 5 | 37 ± 4 | 36 ± 5 | 38 ± 5 | NS |
LVEDVI (mL/m 2 ) | 58 ± 6 | 58 ± 7 | 59 ± 6 | 61 ± 7 | NS |
LVESVI (mL/m 2 ) | 22 ± 4 | 23 ± 5 | 22 ± 4 | 24 ± 5 | NS |
EF (Simpson) (%) | 62 ± 5 | 62 ± 6 | 62 ± 5 | 61 ± 6 | NS |
Fractional shortening (%) | 38 ± 6 | 38 ± 6 | 38 ± 6 | 37 ± 6 | NS |
LVMI (g/m 2 ) | 95 ± 19 | 95 ± 18 | 98 ± 20 | 102 ± 22 | NS |
E (m/sec) | 74 ± 13 | 76 ± 12 | 76 ± 13 | 77 ± 12 | NS |
A (m/sec) | 64 ± 12 | 65 ± 11 | 68 ± 11 | 70 ± 12 | NS |
E/A ratio | 1.2 ± 0.3 | 1.2 ± 0.3 | 1.1 ± 0.3 | 1.1 ± 0.2 | NS |
DT (msec) | 192 ± 22 | 194 ± 25 | 199 ± 25 | 205 ± 26 | NS |
For myocardial tissue velocities and strain and strain rate, analyses of covariance were used to evaluate trends in mean values of BMI-adjusted LV tissue Doppler measures across the groups ( Table 4 ).
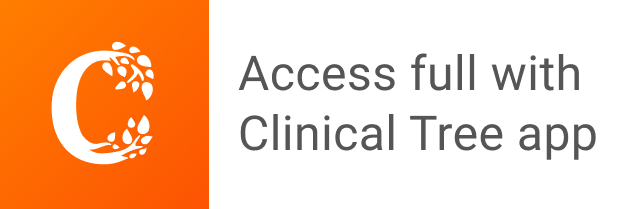