Abstract
Background
Lipoprotein(a) is a unique, atherogenic glycoprotein that was reported in humans more than six decades ago and is an independent risk factor for atherosclerotic cardiovascular disease, including myocardial infarction and stroke.
Aim of review
The purpose of this article is to review lipoprotein(a) biology, associations with disease, treatment, and potential value of screening in pediatrics.
Key scientific concepts of review
Lipoprotein(a) has a lipid-rich core which contains triglycerides and cholesterol esters surrounded by a phospholipid shell containing an apolipoprotein B 100 covalently bound to apolipoprotein(a). Lipoprotein(a) levels increase from birth to age two years and remain stable afterward. Lipoprotein(a) has a six-fold greater association with coronary heart disease than low-density lipoprotein. Type 2 diabetes may be associated with obesity, metabolic dysfunction, and vascular endothelial inflammation, but low lipoprotein(a) levels. In children, there is no association between lipoprotein(a) levels and obesity. Metabolic dysfunction-associated steatotic liver disease may be associated with obesity, metabolic syndrome, diabetes, hypertension, metabolic dysfunction-associated steatohepatitis, hepatic fibrosis, and hepatocellular carcinoma. Patients with metabolic dysfunction-associated steatotic liver disease and low lipoprotein(a) levels may be more likely to develop hepatic fibrosis. Lipoprotein(a) levels may be decreased with some proprotein convertase subtilisin/kexin type 9 inhibitors, small interfering RNAs, and antisense oligonucleotides and lipoprotein apheresis, but not statin drugs. Universal pediatric lipoprotein(a) screening is being considered because it may predict atherosclerotic cardiovascular disease in adulthood.
Graphical abstract
Graphical abstract illustrating the associations observed between high lipoprotein(a) (Lp[a]) levels and atherosclerotic cardiovascular disease (ASCVD). Low Lp(a) levels are associated with type 2 diabetes and hepatic fibrosis. As Lp(a) levels reach stability by age 2 years, it may be beneficial to implement universal pediatric Lp(a) screening. People of African and African American ancestry are at increased risk for having Lp(a) levels >50 mg/dL (Koschinsky et al., 2024; Nissen et al., 2022), and people with increased levels are asymptomatic until they have an ASCVD event.

Highlights
- •
Lipoprotein(a) level is an independent risk factor for atherosclerotic cardiovascular disease.
- •
Lipoprotein(a) reaches adult levels in children by age two years.
- •
Lipoprotein(a) levels have direct and inverse associations with metabolic diseases.
- •
Treatments are being developed to lower elevated lipoprotein(a) levels.
- •
Lipoprotein(a) screening identifies patients at risk for cardiovascular disease.
1
Introduction
In contrast with well-known lipoproteins such as low-density lipoprotein (LDL) and high-density lipoprotein (HDL), testing for the atherogenic lipid particle lipoprotein(a) (Lp[a]) by primary care providers and specialists is limited because of the limited knowledge about Lp(a) and its contribution to atherosclerotic and metabolic disease. However, Lp(a) is receiving attention because of updated evidence about the function of Lp(a) in the pathophysiology of metabolic dysfunction-associated steatotic liver disease (MASLD), including associations between Lp(a) levels in MASLD patients and the development of metabolic dysfunction-associated steatohepatitis (MASH), hepatic fibrosis, and cirrhosis.
Although Lp(a) in humans was reported more than six decades ago [ ], knowledge gaps persist about Lp(a) function and physiology. The growing public awareness about Lp(a) and its role as an independent risk factor for atherosclerotic cardiovascular disease (ASCVD) may create opportunities for ongoing research, education, and advocacy. By facilitating education about this lesser-known lipid particle and advocating for increased testing of Lp(a) levels in patients, we may improve risk stratification, treatment, and health outcomes in patients who have elevated levels.
As novel therapies are developed that are more effective in lowering Lp(a) in tandem with LDL levels, improved protocols are needed for screening Lp(a) levels. Although guidelines from the American Heart Association and National Lipid Association recommend that all people should have Lp(a) levels assessed at least once as adults [ , ], pediatric providers have a complex dilemma. If preventive medicine enables improved health outcomes for our patients, information is needed about the function of Lp(a) in primary, secondary, and primordial prevention of ASCVD and the optimal age to screen Lp(a) levels in children and adolescents. As atherosclerotic disease may begin in early infancy and childhood, pediatric providers need guidance for optimal early identification of asymptomatic patients who are at increased risk for ASCVD. Although universal screening guidelines are recommended by the American Academy of Pediatrics, American Heart Association, American Cardiology Association, and National Lipid Association for testing fasting lipid levels in patients aged 9 to 11 years [ ], there currently is no universally recommended screening guideline for measuring Lp(a) levels in pediatric patients, except in patients having high risk associated with a family history of homozygous familial hypercholesterolemia [ ].
Lp(a) levels begin to increase after birth and continue to increase until a plateau level is reached at age 2 years [ , ]. Lp(a) levels subsequently remain constant, with minimal variation throughout the adult years, because 90 % of Lp(a) expression is determined genetically [ ]. This early achievement of a steady Lp(a) level in children may provide a crucial screening opportunity for pediatric and adult preventive care. As children develop stable adult Lp(a) levels in the first few years after birth, universal Lp(a) screening may provide important opportunities for lifelong cardiometabolic preventive care. When an Lp(a) level of 350 mg/dL (normal range 10–30 mg/dL) is identified in a patient aged 3 years of age instead of 40 years, the patient would have almost 4 decades to engage in primordial and primary prevention, potentially improving outcomes for the patient and the entire family.
Lp(a) levels above 50 mg/dL (125 nmol/L) are associated with increased risk of ASCVD events and are elevated in 20 % to 30 % of the population [ ]. Despite normal HDL and non-HDL cholesterol levels, an elevated Lp(a) level is a well-established risk factor for adverse cardiovascular events, especially when combined with an elevated LDL cholesterol (LDL-C) level [ ]. Therefore, the evidence about the importance of Lp(a) in cardiometabolic health may support the initiation of routine Lp(a) screening during childhood and expansion of universal pediatric lipid screening guidelines to include a global recommendation for measuring an Lp(a) level at least once in childhood.
The purpose of this article is to review the biology of Lp(a), associations between Lp(a) and atherosclerosis, metabolic dysfunction, type 2 diabetes, and liver disease, treatment of elevated Lp(a) levels, and potential value of Lp(a) screening in pediatrics and preventive health.
2
Lipoprotein(a) biochemistry, genetics, and pathophysiology
Lp(a) is a molecular complex that is analogous in structure to LDL ( Fig. 1 ). Lp(a) has a lipid-rich core that contains triglycerides and cholesterol esters and is surrounded by an outer phospholipid shell. The Lp(a) surface contains distinctive proteins such as the apolipoprotein B 100 (apo B 100 ) protein and highly complex apolipoprotein(a) (apo[a]). The apo(a) has several unique domains and multiple triple-looped glycoproteins, which are known as kringle IV domains (KIV) and numbered from type 1 (KIV-1) to 10 (KIV-10), a kringle V domain (KV), and an inactive protease terminal domain [ ]. Although apo(a) contains one copy each of KIV-1, KIV-3 through KIV-10, and KV, the KIV-2 is highly variable in expression.

The KIV-2 is present in varied copy numbers in different people, depending on individual variation in the amount of LPA gene expression [ ]. Each person normally has two LPA gene alleles, one from each parent, resulting in two genetically different versions of intrinsic Lp(a) molecules in each individual. Each of the two subpopulations of Lp(a) has a distinctive copy number of KIV-2 repeats, depending on the inherited allele [ ]. Human Lp(a) may have KIV-2 repeats that vary in number from two copies to >50 copies [ ].
In addition to the highly specified and complex structure of the apo(a) that is covalently bound to apo B 100 on the surface of Lp(a), the apo(a) also contains oxidized phospholipids. Lp(a) is the major lipoprotein carrier of oxidized phospholipids in the body. These oxidized phospholipids cause oxidative injury, endothelial damage, and vascular inflammation that initiates atheroma development [ ]. Therefore, Lp(a) is associated with an increased risk of developing ASCVD and adverse cardiovascular outcomes such as coronary heart disease, myocardial infarction, stroke, and cardiovascular-kidney-metabolic syndrome [ ].
The Norwegian geneticist Kåre Berge discovered Lp(a) in 1963 while performing immunological studies on human serum and showed that Lp(a) follows a genetic inheritance pattern [ ]. By 1974, Berge and colleagues showed that patients with coronary heart disease had a higher frequency of elevated Lp(a) levels than healthy control subjects. It later was discovered that the plasminogen gene ( PLG ) evolved from the Lp(a) gene ( LPA ) through numerous mutations and gene alterations and that Lp(a) may interfere with plasminogen and inhibit fibrinolysis [ ]. In addition to the effects of the oxidized phospholipids of Lp(a), Lp(a) promotes atherothrombosis by inhibiting streptokinase, urokinase, and tissue-type plasminogen activator, which results in preventing the conversion of plasminogen to plasmin [ ]. Lp(a) also increases the risk of developing thromboses by increasing clot stabilization [ ].
3
Lipoprotein(a) in human disease
Although the physiologic purpose of Lp(a) is unknown, Lp(a) levels are associated with human disease. Lp(a) levels are determined more by genetic than environmental factors. Increased Lp(a) levels are associated with higher risks of developing cardiovascular disease, including myocardial infarction, stroke, arrhythmias, valvular heart disease, peripheral artery disease, and thromboembolic disease [ ]. Elevated Lp(a) levels may be observed in 20 % of people [ ], and the risk of developing an acute myocardial infarction is three-fold higher in people with Lp(a) level >50 mg/dL [ ]. Furthermore, elevated Lp(a) levels may be associated with coronary heart disease in 16 % of people and stroke in 10 % of people.
Lp(a) levels may be low in newborns but increase during the first few months after birth. Full LPA gene expression is reached by age two years [ , ]. Children may have minimal fluctuation in Lp(a) levels from age two years throughout their lifetime, except for increased Lp(a) levels after menopause. Children or adolescents with elevated Lp(a) levels typically have no associated clinical symptoms or signs. However, they may have a family medical history of obesity, diabetes, hypertension, or dyslipidemia, which may be undiagnosed in first- or second-degree relatives [ ]. The American Heart Association has recommended Lp(a) screening in pediatric patients with familial hypercholesterolemia because these patients are at high risk of developing premature cardiovascular disease [ ].
Diagnostic strategies, treatment, and risk levels for children of various ethnicities with elevated Lp(a) levels are being evaluated [ , ]. Elevated Lp(a) levels are more commonly observed in African and African American patients [ ]. African American children may have higher Lp(a) levels and associated cardiovascular risks than White children [ ]. African American more than White children have an association between elevated LDL-C and Lp(a) levels [ , ].
Multiple treatments have been evaluated to decrease elevated Lp(a) levels, including physical activity, dietary changes, and medications, but Lp(a) levels may be unchanged or only minimally lowered by these interventions, and some medications, such as statin drugs may increase Lp(a) levels [ ]. As elevated Lp(a) level may be an independent risk factor for ASCVD, the lack of effective treatment strategies creates a dilemma for clinicians and patients after elevated Lp(a) levels are identified.
4
Lipoprotein(a) and atherosclerosis
Atherosclerosis is a progressive inflammatory disease of blood vessels involving lipid-rich plaques that cause major ischemic cardiovascular events such as angina pectoris, myocardial infarction, and stroke [ , ]. Heart disease is the first and stroke is the fifth most common cause of death in the United States [ , ]. The Framingham study and Multiple Risk Factor Intervention Trial (MRFIT) have shown that cholesterol level is a key component in the development of atherosclerosis. Lipoproteins such as LDL and Lp(a) are important in the deposition of cholesterol in affected arteries [ ], and LDL-C is important in the pathogenesis of atherosclerosis.
Endothelial cells of arteries are sensitive to adverse stimuli such as mechanical or oxidative stress. In response to these stimuli, the protective endothelial barrier is lost, and LDL particles and very low-density lipoprotein (VLDL) remnants accumulate on the intima and become oxidized by reactive oxygen species. Endothelial cell activation through oxidative stress or injury causes upregulation of monocyte adhesion molecules and proinflammatory mediators. The increase in oxidized LDL and production of inflammatory cytokines may cause further endothelial cell activation, perpetuating the inflammatory cycle and causing the localization of foam cells and development of fatty streaks and atherosclerotic plaques on vessel walls. Fibrous caps form on the plaques and contribute to thrombus formation and then plaque rupture. HDL provides a rescue mechanism by degrading accumulated LDL adhered to the intima, preventing the formation of fibrous caps on existing plaques, and limiting the amount of smooth muscle apoptosis and elastase degradation that may occur [ ].
In addition to LDL and HDL, Lp(a) has a unique function in the pathophysiology of ASCVD. Lp(a) is more atherogenic per particle than LDL. Variant forms of apo B 100 may be observed between Lp(a) and LDL and used to assess relative atherogenicity. Data from the UK Biobank and CARDIoGRAMplusC4D study showed that Lp(a) had a higher association with coronary heart disease risk than LDL [ ]. The relative risk for coronary heart disease between Lp(a) apo B 100 versus LDL apo B 100 per unit increase in particle number was 6.6 for the UK Biobank data and 3.8 for the CARDIoGRAMplusC4D study [ ]. Furthermore, the hazard ratio for coronary heart disease per 50 nmol/L (∼ 23.3 mg/dL) increase in apo B 100 was higher for Lp(a) (1.47) than LDL (1.04) [ ]. This study showed a more than six-fold greater association between coronary heart disease risk for Lp(a) than LDL. In response to the observed increased atherogenicity of Lp(a), the relative benefit of strategies to decrease Lp(a) versus LDL level is under evaluation. A decrease in Lp(a) level by greater than or equal to 85 nmol/L (∼ 39.5 mg/dL) may be necessary to achieve the same risk reduction (22 %) as a decrease in LDL-C by 1 mmol/L in a five-year clinical trial [ ]. Therefore, Lp(a) is highly atherogenic, and Lp(a) levels >108 nmol/L or 50 mg/dL is a risk factor for the development of ASCVD.
The mechanism behind Lp(a) as a contributor to ASCVD is incompletely understood. The increased atherogenicity of Lp(a) compared to LDL-C may be associated with the preferential carrying capacity of Lp(a) for apo(a) with multiple oxidized phospholipids [ ]. The oxidized phospholipids may trigger a complex inflammatory cascade that may cause the development of atherosclerosis.
Increased Lp(a) levels are strongly correlated with accelerated progression of low-attenuation plaque formation, which is a strong indicator of plaque instability and a predictor of myocardial infarction [ ]. The oxidized phospholipids may induce proinflammatory pathways by the apo(a) carried by Lp(a), resulting in apoptosis and necrosis in the lipid core of the plaque [ ].
5
Lipoprotein(a) and type 2 diabetes
Although elevated Lp(a) levels are associated with the development of ASCVD, an inverse association is observed between Lp(a) levels and type 2 diabetes. In a prospective study of 26,746 healthy US women who were followed for 13 years to assess the association between type 2 diabetes and baseline Lp(a) levels [ ], there was a 20 % to 50 % lower relative risk of type 2 diabetes observed in the second to fifth quintiles of Lp(a) level versus the lowest quintile [ ]. The mechanism of this association is unknown. However, Lp(a) levels are decreased in patients with hyperinsulinemia, and insulin may decrease Lp(a) levels by suppressing apo(a) production and function in hepatocytes [ ]. Lp(a) plasma concentration is also decreased in type I diabetes patients receiving insulin therapy [ ]. Although obesity is associated with type 2 diabetes, Lp(a) levels in children are not associated with body mass index [ ]. Further research is needed to evaluate the mechanisms causing the inverse association between Lp(a) levels and type 2 diabetes.
6
Lipoprotein(a) and metabolic dysfunction-associated steatotic liver disease
MASLD, which previously was known as nonalcoholic fatty liver disease (NAFLD), is the most common liver disease in children and adolescents and may affect 30 % of adults globally. In MASLD, hepatic steatosis occurs in response to metabolic derangements, including insulin resistance and lipotoxicity caused by increases in excess free fatty acids in hepatocytes [ ]. Chronic hepatocellular injury progresses with cytoplasmic engorgement or ballooning of hepatocytes [ ], which is a key histologic finding on liver biopsy ( Fig. 2 ). In addition, mitochondrial damage, endoplasmic reticulum deterioration and lysosomal dysfunction occur as the disease progresses to MASH, apoptosis, fibrosis, and cirrhosis [ ]. Patients have an increased risk of developing hepatocellular carcinoma [ ].

The nomenclature for steatotic liver disease was reclassified recently from NAFLD to MASLD in a multi-society Delphi consensus statement to refine the diagnosis and diagnostic criteria [ ] and discontinue the use of words such as fatty, which may have negative connotations [ ]. This consensus statement was published by several liver disease associations and endorsed by >70 international medical and pediatric societies [ ]. The updated nomenclature may enable a more descriptive and specific explanation of the metabolic derangements that occur in the liver from abnormal lipid storage, increased inflammation, and increased insensitivity to insulin. It also provided a new description of metabolic dysfunction-associated alcoholic liver disease that is related to metabolic dysfunction yet overlaps with and is more consistent with alcohol predominant hepatic injury. The name change from NAFLD to MASLD emphasizes that this liver disease may involve extrahepatic organs, and MASLD may be the hepatic manifestation of metabolic syndrome [ , ].
The global prevalence of MASLD has increased from 25 % (1990–2006) to 38 % (2016–2019) [ ]. MASLD is a complex disease associated with numerous comorbid conditions, including obesity, metabolic syndrome, diabetes, hypertension, MASH, hepatic fibrosis, and hepatocellular carcinoma [ , ]. A meta-analysis and systematic review of the global prevalence of MASLD and obesity showed that 75 % of patients with obesity had concomitant MASLD [ ]. A meta-analysis of the global rates of pediatric MASLD from 1997 to 2023 showed that MASLD prevalence was 13 % in non-obese patients and 47 % in obese patients [ ].
There are strong associations between the development of MASLD, obesity, and type 2 diabetes. A meta-analysis globally showed that the pooled prevalence of type 2 diabetes in MASLD (NAFLD) patients was 28.3 % (95 % confidence interval, 25.2 % to 31.6 %) [ ]. Another meta-analysis of 129 studies showed a significantly greater risk of adverse cardiovascular outcomes, chronic kidney disease, prediabetes, type 2 diabetes, metabolic syndrome, and hypertension in patients with than without MASLD and a higher risk of type 2 diabetes in patients with severe versus less severe MASLD [ ]. More than half of all type 2 diabetes patients globally may have MASLD (55.5 %; 95 % confidence interval 47.3 %–63.7 %) [ ]. Furthermore, MASLD is associated with a higher incidence of portal vein thrombosis than other liver diseases [ ], and 24.4 % of patients with inflammatory bowel disease globally also may have MASLD [ ].
MASLD may be associated with cardiovascular disease because both conditions are associated with obesity and type 2 diabetes. As liver dysfunction develops with the metabolic stress associated with abnormal glucose regulation, insulin resistance, and lipid metabolism, atherosclerosis also develops from oxidative stress, vascular inflammation, and plaque formation [ , ]. The marked inflammation and cytokine-mediated responses induced by MASLD, with increased LDL and decreased HDL availability for reuptake of LDL from plaques, may perpetuate the progression of atherosclerosis [ , ].
There are associations between obesity and the risk of developing type 2 diabetes and MASLD. However, Lp(a) levels in children are not associated with obesity [ ], elevated Lp(a) levels are associated inversely with the diagnosis of type 2 diabetes [ ], and a correlation between the incidence of MASLD and low Lp(a) levels [ ]. Although obese and type 2 diabetes patients may have higher levels of LDL-C and lipoproteins containing apo B, which include LDL and Lp(a), it is unknown whether there may be a direct correlation between increased Lp(a) level and MASLD. Further research is justified to clarify the relationship between Lp(a) levels and MASLD.
7
Lipoprotein(a) and hepatic fibrosis in MASLD
Lp(a) is associated with atherosclerotic plaque formation and is a greater cardiovascular risk factor than LDL. Although Lp(a) resembles LDL structurally, the physiologic function of Lp(a) is unclear [ ]. Yet, 20 % of people globally have elevated Lp(a) levels. As Lp(a) is associated with the development of cardiovascular disease and atherosclerosis, possible associations between Lp(a) levels, MASLD, and the development of hepatic fibrosis may be important. However, studies assessing the associations between MASLD and Lp(a) have had mixed results [ ]. There may be an inverse relationship between Lp(a) levels and MASLD-associated liver fibrosis, and patients who develop MASLD-associated fibrosis may have low Lp(a) levels [ ]. The association between low Lp(a) and increased transaminase levels may be a possible biomarker to predict the development of advanced fibrosis with MASLD [ ].
As Lp(a) is produced in the liver, diseases resulting in impaired liver function may be associated with low Lp(a) levels that correlate with liver disease progression [ ]. Therefore, the observed decreased levels of Lp(a) in patients with MASLD may confound the use of Lp(a) level as a diagnostic tool for ASCVD. Furthermore, MASLD with advanced hepatic fibrosis may skew Lp(a) levels and decrease the potential that Lp(a) levels may identify the risk of carotid atherosclerosis [ ]. Although the connection between MASLD and Lp(a) levels in ASCVD and other cardiovascular disease is unclear, further research is justified to evaluate the relationships between Lp(a), MASLD, and ASCVD and potential use of Lp(a) in clinical diagnosis and treatment.
As MASLD progresses, 30 % to 40 % of MASLD patients develop MASH, which is manifested by a persistent inflammatory state, and 74 % of MASH patients advance to more severe liver disease, including hepatic fibrosis and cirrhosis [ , ]. Hepatic fibrosis is characterized by the accumulation of extracellular matrix proteins, such as collagen, because of prolonged inflammation. The distribution of fibrosis deposition varies with the underlying source of the liver injury. In MASH, pericellular and perisinusoidal fibrosis deposition may occur in the centrilobular areas of the liver.
Hepatic stellate cells, also known as perisinusoidal cells, are quiescent in the healthy liver but become activated after liver injury. Activation of hepatic stellate cells transforms them into proliferative myofibroblasts that contribute to extracellular matrix accumulation and fibrosis in patients with MASLD and MASH [ ]. Hepatic stellate cells are the principal contributors of fibrogenesis in MASLD and MASH, generating 80 % to 95 % of the collagen-producing myofibroblasts [ ].
In MASLD and MASH, hepatic steatosis causes elevated levels of free fatty acids in the liver. These excess free fatty acids stimulate the production of reactive oxygen species and associated oxidative stress, which perpetuates the progression of fibrosis. Oxidative stress may inhibit mature hepatocyte replication, resulting in the accumulation of immature progenitor cells, the formation of small bile ductules, and fibrosis progression, facilitated by the release of proinflammatory cytokines and the differentiation of hepatocytes into fibrogenic myofibroblasts [ ].
Several factors contribute to the development and progression of hepatic fibrosis in MASLD. Patients with advanced fibrosis in MASLD may have features of metabolic syndrome, such as central obesity, insulin resistance, and dyslipidemia. Insulin resistance and hyperinsulinemia exacerbate hepatic lipid accumulation, inflammation, injury, and fibrogenesis [ ]. In addition, polymorphisms in genes involved in lipid metabolism and fibrogenesis may predispose individuals to a higher risk of developing hepatic fibrosis in MASLD and cause variation in fibrosis severity between individuals [ , ]. The variation in hepatic fibrosis severity in MASLD may be affected by clinical and demographic factors such as age, ethnicity, hormonal status, and sex. Postmenopausal women and men have a higher risk of advanced fibrosis than premenopausal women, suggesting that estrogen may be protective against fibrogenesis.
MASLD is associated with increased susceptibility to ASCVD. This association is complex and stems from several pathways. Hepatic steatosis is associated with the release of proinflammatory cytokines, which accelerate the formation of plaque and endothelial dysfunction in arteries. Effective dilation of large and small arteries may depend on the capacity of the endothelium to produce nitric oxide, which regulates blood flow by causing vasodilation, but inflammation may compromise nitric oxide production [ ]. In addition, disrupted glucose metabolism and hepatic insulin resistance in MASLD may contribute to ASCVD pathogenesis. The irregularities in glucose regulation in MASLD arise from systemic inflammation, visceral obesity, and accumulation of dysfunctional ectopic fat tissue [ ]. Systemic inflammation, characterized by proinflammatory cytokine production and oxidative stress, may contribute to worsening insulin resistance, which may cause increased lipolysis in adipose tissue, uptake of non-esterified fatty acids by hepatocytes, and synthesis of triglycerides in the liver [ ].
The increased fatty acids in the liver result in mitochondrial and peroxisomal β-oxidation, which may generate more reactive oxygen species. In addition, the release of proinflammatory cytokines may stimulate the production of suppressors of cytokine signaling proteins. Elevated levels of cytokine suppressors in the liver may increase hyperinsulinemia and insulin resistance [ ]. Furthermore, MASLD is associated with atherogenic dyslipidemia, characterized by elevated levels of LDL-C, triglycerides, and apo B, decreased HDL cholesterol levels, and increased ASCVD risk. This dyslipidemic profile arises from ongoing impaired lipid metabolism that persists because of insulin resistance, increased hepatic triglyceride synthesis, and decreased clearance of triglyceride-rich lipoproteins from the liver [ ].
Glucagon-like peptide 1 agonists are being evaluated in treating MASLD and may limit the development of hepatic fibrosis. These medications may improve insulin resistance in MASLD by increasing glucose-dependent insulin secretion and improving insulin sensitivity [ ]. They cause satiety and decreased food intake, resulting in weight loss and improved metabolism [ ]. Furthermore, these medications may inhibit hepatic lipogenesis, decrease circulating free fatty acids, and increase mitochondrial turnover and oxidative capacity, which may decrease liver fat accumulation and inflammation and prevent the transition from steatotic liver disease to steatohepatitis [ ]. Glucagon-like peptide 1 agonists also exert anti-inflammatory effects by inhibiting hepatocyte apoptosis and intrahepatic inflammatory responses, attenuating the progression of hepatic injury and fibrosis [ ]. Clinical trials have shown decreases in liver fat content and improvements in liver histology in patients with MASLD and MASH after treatment with glucagon-like peptide 1 agonists [ ].
8
Treatment for elevated lipoprotein(a) levels
An absolute reduction in Lp(a) level of approximately 100 mg/dL in adults that have an Lp(a) level >90 to 100 mg/dL may decrease coronary heart disease events by 15 % to 20 %, according to a mendelian randomization analysis, and clinical benefit may be proportionate to the reduction in Lp(a) level [ ]. However, aside from plasmapheresis in high-risk individuals after myocardial infarction, treatments for elevated Lp(a) levels may have limited efficacy, and none are approved by the United States Food and Drug Administration for this clinical indication alone [ ]. There is even less information available about the effects of lowering Lp(a) levels in children and adolescents. Therefore, larger studies of longer duration are needed in children and adults to provide more robust data [ ]. The first-line therapy for most pediatric lipid disorders typically includes lifestyle modification and diet, which may cause weight loss and improved LDL and HDL levels but may not decrease Lp(a) levels [ ].
Statin therapy does not decrease Lp(a) levels, possibly because the LDL receptor may have limited or no function in clearing Lp(a) [ ]. Statin drug use may be associated with increased Lp(a) levels and poorer cardiovascular outcomes compared with placebo. High Lp(a) levels (≥ 50 mg/dL) were observed at both baseline and during follow-up for patients treated with statins [ ]. Most current research has shown that statin therapy does not affect Lp(a) levels [ ]. Although guidelines recommend statin therapy in adults aged 40 to 75 years without diabetes who have an intermediate 10-year ASCVD risk of heart attack or stroke (7.5 % to 19.9 %) or borderline risk (5 % to 7.4 %) or Lp(a) level equal to or >50 mg/dL [ ], the statin medication primarily may decrease LDL levels and risk factors of developing ASCVD other than the Lp(a) level. Furthermore, various lipid-lowering strategies, including statin drugs in combination with ezetimibe or niacin, may increase Lp(a) levels by 11 % [ ].
Other medications tested for lowering Lp(a) levels have shown no overall benefit. Niacin was associated with an Lp(a) reduction of 30 % in two clinical trials, but detrimental adverse effects were observed [ ]. Furthermore, these trials were not designed for patients with high Lp(a) levels. In the AIM-HIGH trial, patients had a mean pre-treatment Lp(a) level of 36 nmol/L (∼16.4 mg/dL), and niacin improved LDL-C levels but did not change ASCVD outcomes; niacin was associated with mean Lp(a) level decrease of 21 % but did not decrease the ASCVD event rate in patients with high Lp(a) levels (>50 mg/dL) [ ].
Proprotein convertase subtilisin/kexin type 9 (PCSK9) inhibitors may decrease Lp(a) levels by 15 % to 26 %. The PCSK9 inhibitor evolocumab may decrease Lp(a) levels by 26.9 %, independent of LDL-C levels [ ]. A pooled analysis of four phase 2 trials with 1359 patients showed significant dose-related decreases in Lp(a) level with evolocumab compared with placebo, with evolocumab 140 mg every two weeks decreasing Lp(a) level by 29.5 % and 420 mg every four weeks by 24.5 % [ ].
In another trial, the PCSK9 inhibitor alirocumab decreased the risk of major adverse cardiovascular events by 30 % in patients with LDL-C levels <70 mg/dL, but this reduction was significant only when Lp(a) levels were greater than the median level (13.7 mg/dL) [ ]. A pooled analysis from three phase 2 trials showed that alirocumab (150 mg every two weeks) decreased Lp(a) levels by 30 % compared with placebo after 8 to 12 weeks, with consistent decreases noted for various ranges of pre-treatment Lp(a) values [ ]. The most marked absolute decreases were noted in patients with higher initial Lp( a )levels, and the decrease in Lp(a) level was not correlated with the decrease in LDL-C level [ ].
Inclisiran, which is a small interfering RNA (siRNA) that blocks the synthesis of PCSK9, may have prolonged effects in decreasing LDL-C level after a single dose but does not decrease Lp(a) level [ ]. A single dose of olpasiran, which is a siRNA designed to target Lp(a) mRNA, was associated with a dose-dependent decrease in mean Lp(a) level compared with placebo in adults with Lp(a) levels equal to or >70 nmol/L (∼32.5 mg/dL).
In contrast with the effects of statin drugs on LDL levels, the mechanism by which PCSK9 inhibitors decrease Lp(a) levels is unknown [ ]. In a study of Chinese patients, no association was observed between levels of PCSK9 and Lp(a), suggesting that PCSK9 inhibitors may decrease Lp(a) through a mechanism not directly related to the PCSK9 pathway.
Cholesteryl ester transfer protein inhibitors, thyroid hormone analogs, or microsomal triglyceride transfer protein inhibitors may have effects on decreasing Lp(a) levels. Cholesteryl ester transfer protein inhibitors also may lower LDL-C levels. However, randomized trials of three cholesteryl ester transfer protein inhibitors, torcetrapib, evacetrapib, and dalcetrapib, were terminated prematurely because of toxicity or futility [ ].
Lipoprotein apheresis is highly effective at lowering Lp(a) levels temporarily [ ]. Apheresis may cause a time-averaged 30 % to 35 % decrease in Lp(a) levels but may be impractical because long-term therapy may require two- to three-hour sessions, once or twice per week, for the lifespan of the patient. Apheresis may decrease LDL-C and Lp(a) levels in patients with Lp(a) levels greater than the 95th percentile and decrease ASCVD risk [ ]. However, studies performed to date had no simultaneous control group, and outcomes of patients who had apheresis were compared with historical controls [ ]. In addition, proper interpretation of results is difficult because most sub-studies of Lp(a) levels that evaluated other medications did not recruit patients with elevated Lp(a) levels and were post hoc analyses of patients having low Lp(a) levels.
Antisense oligonucleotides (ASOs) are small molecules and antibodies that inhibit enzyme activity or receptor proteins. The development of ASOs may represent a turning point in Lp(a) research, and randomized trials have evaluated ASOs in patients with elevated Lp(a) levels [ ]. In apo(a) transgenic mice, an ASO specific to apo(a) decreased circulating apo(a) levels by 86 % without affecting apo B [ ]. Optimized ASOs to apo(a) have been evaluated in humans [ ], and three randomized clinical trials showed that increasing ASO doses correlated with greater decreases in mean Lp(a) [ ]. Mipomersen, which is a second-generation ASO targeting apo B 100 , has been approved in the United States for treating homozygous familial hypercholesterolemia and has been associated with a mean absolute reduction in Lp(a) of 11 mg/dL (−17 %) after at least 12 months of treatment [ ].
Pelacarsen is an ASO that contains a conjugated triantennary N -acetyl galactosamine molecule. Pelacarsen was designed to be highly and selectively taken up by hepatocytes through the interaction of the triantennary N -acetyl galactosamine with the hepatocyte asialoglycoprotein receptor [ ]. This ASO was evaluated in 47 healthy adults who had an Lp(a) level equal to or >25 nmol/L (∼ 11.6 mg/dL) in a randomized, double-blind, placebo-controlled dose-ranging trial and showed that pelacarsen was associated with significant dose-dependent decreases in Lp(a) levels. [ , ]. The ASO conjugated to triantennary N -acetyl galactosamine enabled a decrease in Lp(a) levels by 99 % at a tolerable dose. This was a major improvement over previous drugs that decreased Lp(a) levels by only 40 % because a 40 % decrease was insufficient for achieving therapeutic benefit in patients with extremely high Lp(a) levels. Although pelacarsen is effective in lowering Lp(a) levels, it requires high doses to penetrate hepatocytes that synthesize apo(a), raising concerns for the potential of drug-related toxicities, as well as flu-like symptoms and injection site reactions [ ].
9
Conclusion: lipoprotein(a) in pediatrics
In summary, Lp(a) is a complex lipoprotein that is being increasingly recognized for its importance in cardiovascular disease. Despite extensive research in adults, limited information is available about evaluating and treating Lp(a) levels in children and adolescents. As Lp(a) levels are predominantly determined by genetic factors [ ], long-term sustained preventive healthcare programs potentially may be guided by the identification of elevated Lp(a) levels in family members.
Pediatric studies show that Lp(a) levels are stable from early childhood through adolescence, and high Lp(a) levels are an early marker of atherosclerosis. Children with elevated Lp(a) levels have increased carotid intima-media thickness and arterial stiffness, which are precursors of cardiovascular disease [ ]. Children with familial hypercholesterolemia may present with high Lp(a) levels, which may further compound cardiovascular risk. The presence of high LDL-C and Lp(a) levels in these children necessitates early and aggressive treatment to lower the risk of developing future cardiovascular events [ ].
Treatment of elevated Lp(a) levels in children may be a major challenge for pediatric providers because conventional lipid-lowering therapies, such as statin drugs, have minimal effect on Lp(a) levels [ ]. Although limited data are available in children and adolescents, the use of PCSK9 inhibitors may benefit children with high Lp(a) levels, especially children with familial hypercholesterolemia [ ]. An early-phase clinical trial in adults showed that an ASO medication may be associated with major decreases in Lp(a) levels [ ]. Further research is justified in evaluating the safety and efficacy of PCSK9 inhibitors and other newer therapies, such as ASOs and siRNA medications in children and adolescents.
Gaps in pediatric Lp(a) research also include the limited availability of epidemiologic and longitudinal studies. Such studies are essential to characterize the natural history associated with elevated Lp(a) levels from childhood into adulthood and long-term cardiovascular outcomes associated with high Lp(a) levels in children. Children, adolescents, and young adults who have elevated Lp(a) levels (≥ 30 mg/dL) have a two- to 2.5-fold greater risk of developing ASCVD in adulthood than individuals with normal Lp(a) levels [ ]. Although healthy lifestyles that are started in childhood and maintained throughout life may provide overall health benefits and improve other risk factors associated with ASCVD, these lifestyles may not improve elevated Lp(a) levels [ ].
There is no current consensus about the optimal age for screening or cutoff values of elevated Lp(a) levels that would indicate the advisability of treatment in children. Therefore, further research and standardized guidelines are needed for screening and treating elevated Lp(a) levels in children and adolescents. Establishing evidence-based guidelines may facilitate early identification and intervention and potentially decrease long-term cardiovascular risk [ ]. Furthermore, the development of universal pediatric screening guidelines for Lp(a) levels in children may promote major shifts in public health toward successful implementation of primordial and primary prevention for atherosclerotic cardiometabolic diseases and provide informed risk stratification for high-risk patients. The longitudinal results showing a strong association between elevated childhood Lp(a) levels and adult ASCVD provide evidence in favor of universal Lp(a) screening in childhood [ , ].
Artificial intelligence declaration
AI was not used in preparation of this manuscript.
CRediT authorship contribution statement
Kathryn L. Williams: Conceptualization, Supervision, Writing – original draft, Writing – review & editing, Validation. Maya Augustine: Validation, Writing – original draft, Writing – review & editing. Eru Sujakhu: Validation, Writing – original draft, Writing – review & editing. Justine Magadia: Validation, Writing – original draft, Writing – review & editing. Lindsay Crawford: Validation, Writing – original draft, Writing – review & editing. Aimee Knott: Validation, Writing – original draft, Writing – review & editing. Skyler Hamilton: Validation, Writing – original draft, Writing – review & editing. Uzoma Obiaka: Supervision, Validation, Writing – review & editing.
Funding
Editorial support was provided by the Dean’s Office, University of South Alabama, Frederick P. Whiddon College of Medicine.
Editorial Footnote
Given the role of Guest Editor, UzomaObiaka, had no involvement in the peer-review of this article and has no access to information regarding its peer-review. Full responsibility for the editorial process for this article was delegated to the Managing Editor, Miriam Mestre.
Declaration of competing interest
The authors have no competing interests to disclose.
Acknowledgements
The authors thank Benjamin Estrada, Richard Honkanen, Wei Xin, and Kathryn Williams for the graphical abstract and figures. We are grateful to Dr. Gul Dadlani for his critical review and John V. Marymont, Emily Wilson, and Elly Trepman for editorial support.
References
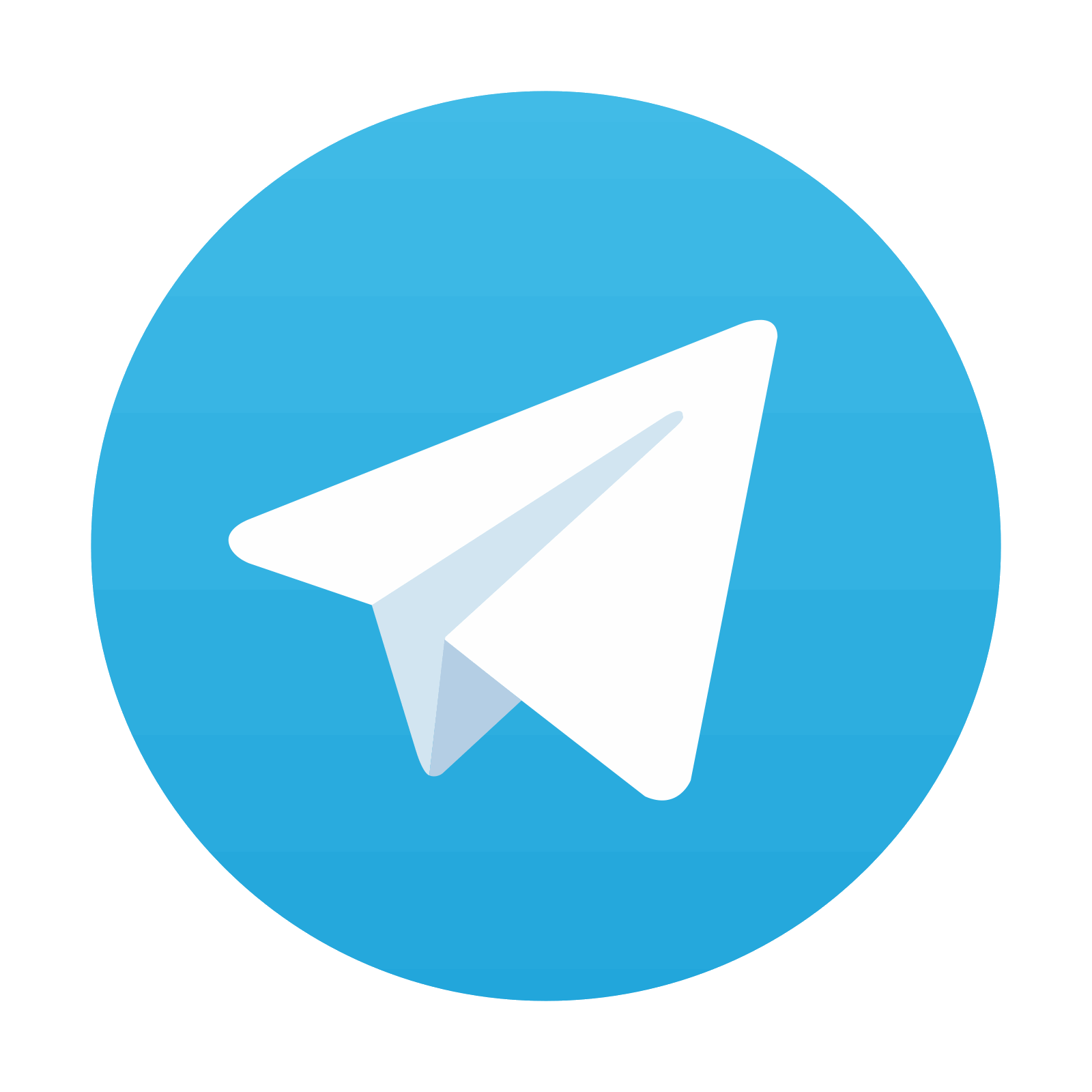
Stay updated, free articles. Join our Telegram channel
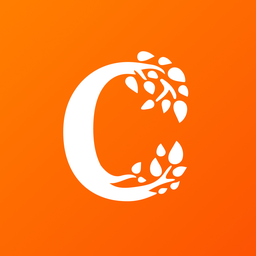
Full access? Get Clinical Tree
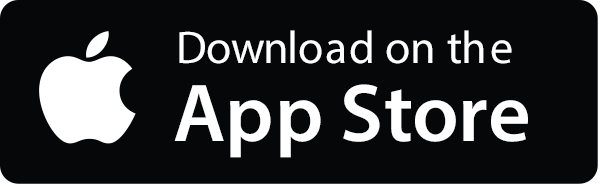
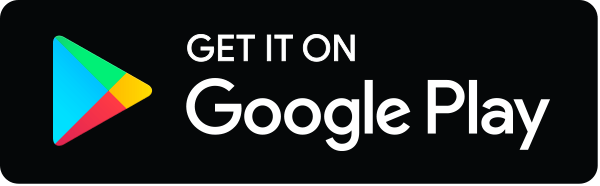
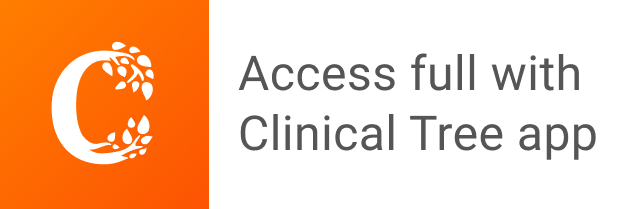