Abstract
Plasma levels of triglycerides (TGs), low-density lipoprotein cholesterol (LDL-C), and high-density lipoprotein cholesterol (HDL-C) are highly heritable traits. The study of their human genomics has identified >150 genes that participate in the regulation of lipoprotein metabolism. A number of Mendelian disorders of lipoprotein metabolism have been molecularly characterized and increasingly merit a personalized approach to diagnosis and treatment. Furthermore, unbiased genome-wide association studies and exome-sequencing studies have yielded new insights into novel pathways, involved in lipid and lipoprotein metabolism, and in some cases have identified new therapeutic targets. Mendelian randomization approaches have confirmed the causal relationship of LDL and TG-rich lipoproteins—and brought into question the causal role of HDL—to atherosclerotic cardiovascular disease. Lipoprotein disorders and plasma lipid traits are increasingly relevant to the clinical application of genomic and personalized medicine.
Keywords
Lipoprotein, apolipoprotein, cholesterol, triglycerides, low-density lipoprotein, high-density lipoprotein, chylomicron, coronary heart disease, genome-wide association study
Chapter Outline
Introduction 27
Overview of Lipoprotein Metabolism 28
Genomics of Plasma Lipids 32
Mendelian Disorders of Lipoprotein Metabolism 32
Mendelian Disorders Causing Elevated Triglyceride Levels 33
Mendelian Disorders Causing Elevated LDL-C Levels 35
Mendelian Conditions Causing Reduced LDL-C Levels 36
Abetalipoproteinemia 36
Familial Hypobetalipoproteinemia 37
PCSK9 Deficiency 37
Familial Combined Hypolipidemia (ANGPTL3 Deficiency) 38
Mendelian Conditions Causing Extreme HDL-C Levels 38
Variants in Lipid-Associated Genes and their Relationship to Cardiovascular Disease 40
LDL-C Variants and CAD 41
TG Variants and CAD 41
HDL-C Variants and CAD 42
Summary and Future Directions 43
References
Introduction
Plasma lipoproteins transport triglycerides (TGs) and cholesterol in the blood and are integral to energy and cholesterol metabolism. However, genetic disorders involving lipoprotein metabolism can predispose to atherosclerotic vascular disease (ASCVD) and its most common manifestation coronary artery disease (CAD). Genetic factors play an important role in influencing lipoprotein metabolism and therefore plasma lipid levels [TGs, low-density lipoprotein cholesterol (LDL-C), high-density lipoprotein cholesterol (HDL-C)] and cardiovascular risk. Molecular and phenotypic characterization of Mendelian lipoprotein disorders has provided major insights into the physiology of lipoprotein metabolism. Unbiased common variant association studies (CVAS) and rare variant association studies (RVAS) have identified new genes and pathways that regulate lipoprotein metabolism and have enhanced our understanding of the relationship between plasma lipids and ASCVD. Lipoprotein metabolism is a ripe area for the application of genomic medicine because of the frequency with which plasma lipids are measured in clinical practice, the quantitative importance of genetics in determining their levels, the large number of gene products involved in lipoprotein metabolism, the relative frequency of some of the Mendelian disorders, and the broad clinical relevance of lipoproteins to ASCVD, the most important cause of morbidity and mortality in the world.
Overview of Lipoprotein Metabolism
Lipoproteins are large macromolecular complexes that transport hydrophobic lipids (primarily TGs, cholesterol, and fat-soluble vitamins) through body fluids (plasma, interstitial fluid, and lymph) to and from tissues. Lipoproteins play an essential role in the absorption of dietary cholesterol, long-chain fatty acids, and fat-soluble vitamins; the transport of TGs, cholesterol, and fat-soluble vitamins from the liver to peripheral tissues; and the transport of cholesterol from peripheral tissues to the liver. Lipoproteins contain a core of hydrophobic lipids (TGs and cholesteryl esters) surrounded by hydrophilic lipids (phospholipids, unesterified cholesterol) and proteins that interact with body fluids. The plasma lipoproteins are divided into five major classes based on their relative density: chylomicrons, very-low-density lipoproteins (VLDLs), intermediate-density lipoproteins (IDLs), low-density lipoproteins (LDLs), and high-density lipoproteins (HDLs). Each lipoprotein class comprises a family of particles that vary slightly in density, size, migration during electrophoresis, and protein composition. The density of a lipoprotein is determined by the amount of lipid per particle. HDL is the smallest and most dense lipoprotein, whereas chylomicrons and VLDL are the largest and least dense lipoprotein particles. Most plasma TG is transported in chylomicrons or VLDL, and most plasma cholesterol is carried as cholesteryl esters in LDL and HDL.
The proteins associated with lipoproteins, called apolipoproteins, are required for the assembly, structure, and function of lipoproteins. Apolipoproteins activate enzymes important in lipoprotein metabolism, and act as ligands for cell surface receptors. ApoA-I, which is synthesized in the liver and intestine, is found on virtually all HDL particles. ApoA-II is the second most abundant HDL apolipoprotein, and is on approximately two-thirds of all HDL particles. ApoB is the major structural protein of chylomicrons, VLDL, IDL, and LDL; one molecule of apoB, either apoB-48 (chylomicrons) or apoB-100 (VLDL, IDL, or LDL), is present on each lipoprotein particle. The human liver synthesizes apoB-100 and the intestine makes apoB-48, which is derived from the same gene by mRNA editing. ApoE is present in multiple copies on chylomicrons, VLDL, and IDL, and plays a critical role in the metabolism and clearance of TG-rich particles. ApoC-II, apoC-III and apoA-V also participate in the metabolism of TG-rich lipoproteins.
The exogenous pathway of lipoprotein metabolism involves the absorption and transport of dietary lipids to appropriate sites within the body ( Fig. 3.1 ). Dietary TGs are hydrolyzed by lipases within the intestinal lumen and emulsified with bile acids to form micelles. Dietary cholesterol, fatty acids, and fat-soluble vitamins are absorbed in the proximal small intestine. Cholesterol and retinol are esterified (by the addition of a fatty acid) in the enterocyte to form cholesteryl esters and retinyl esters, respectively. Longer-chain fatty acids (>12 carbons) are incorporated into TGs and packaged with apoB-48, cholesteryl esters, retinyl esters, phospholipids, and cholesterol to form chylomicrons. Nascent chylomicrons are secreted into the intestinal lymph and are delivered via the thoracic duct directly to the systemic circulation, where they are extensively processed by peripheral tissues before reaching the liver. Lipoprotein lipase (LPL) is synthesized by adipocytes and cardiac and skeletal myocytes and is transported to the capillary endothelial surface, where it is anchored to GPIHBP1 and proteoglycans. The TGs of chylomicrons are hydrolyzed by LPL, and free fatty acids are released; apoC-II, which is transferred to circulating chylomicrons from HDL, acts as a cofactor for LPL in this reaction, and apoA-V also promotes the activity of LPL. A number of circulating proteins, such as apoC-III, ANGPTL3, and ANGPTL4, serve to inhibit the activity of LPL. After hydrolysis of TGs by LPL, the released free fatty acids are taken up by adjacent myocytes or adipocytes and are either oxidized to generate energy or reesterified and stored as TG. The chylomicron particle progressively shrinks in size as the hydrophobic core is hydrolyzed and the hydrophilic lipids (cholesterol and phospholipids) and apolipoproteins on the particle surface are transferred to HDL, creating chylomicron remnants. Chylomicron remnants are rapidly removed from circulation by the liver through a process that requires apoE as a ligand for receptors in the liver.

The endogenous pathway of lipoprotein metabolism refers to the hepatic secretion of apoB-containing lipoproteins and their metabolism ( Fig. 3.2 ). VLDL particles resemble chylomicrons in protein composition, but contain apoB-100 rather than apoB-48 and have a higher ratio of cholesterol to TG (~1 mg of cholesterol for every 5 mg of TG). The TGs of VLDL are derived predominantly from the esterification of long-chain fatty acids in the liver. The packaging of hepatic TGs with the other major components of the nascent VLDL particle (apoB-100, cholesteryl esters, phospholipids, and vitamin E) requires the action of the enzyme microsomal TG transfer protein (MTP). After secretion into the plasma, VLDL acquires multiple copies of apoE and apolipoproteins of the C series by transfer from HDL. As with chylomicrons, the TGs of VLDL are hydrolyzed by LPL, especially in muscle and adipose tissue. After the VLDL remnants dissociate from LPL, they are referred to as IDL, which contain roughly similar amounts of cholesterol and TG. The liver removes approximately 40%–60% of IDL by LDL receptor-mediated endocytosis via binding to apoE. The remainder of the IDL is remodeled by hepatic lipase (HL) to form LDL; during this process most of the TG in the particle is hydrolyzed and all apolipoproteins except apoB-100 are transferred to other lipoproteins. The cholesterol in LDL accounts for more than half of the plasma cholesterol in most individuals. Approximately 70% of circulating LDL is cleared by LDL receptor-mediated endocytosis in the liver.

HDL metabolism is complex ( Fig. 3.3 ). Nascent HDL particles are synthesized by the intestine and the liver. Newly secreted apoA-I rapidly acquires phospholipids and unesterified cholesterol from its site of synthesis (intestine or liver) via efflux promoted by the membrane protein ATP (adenosine triphosphate)-binding cassette (ABC) protein A1 (ABCA1). This process results in the formation of discoidal HDL particles, which then recruit additional unesterified cholesterol from the periphery. Within the HDL particle, the cholesterol is esterified by lecithin-cholesterol acyltransferase (LCAT), a plasma enzyme associated with HDL, and the more hydrophobic cholesteryl ester moves to the core of the HDL particle. As HDL acquires more cholesteryl ester, it becomes spherical, and additional apolipoproteins and lipids are transferred to the particles from the surfaces of chylomicrons and VLDL during lipolysis. HDL-C is transported to hepatoctyes by both an indirect and a direct pathway. HDL cholesteryl esters can be transferred to apoB-containing lipoproteins in exchange for TG by the cholesteryl ester transfer protein (CETP). The cholesteryl esters are then removed from the circulation by LDL receptor-mediated endocytosis. HDL-C can also be taken up directly by hepatocytes via the scavenger receptor class BI (SR-BI), a cell surface receptor that mediates the selective transfer of lipids to cells. HDL particles undergo extensive remodeling within the plasma compartment by a variety of lipid transfer proteins and lipases. The phospholipid transfer protein has the net effect of transferring phospholipids from other lipoproteins to HDL. After CETP-mediated lipid exchange, the TG-enriched HDL becomes a much better substrate for HL, which hydrolyzes the TGs and phospholipids to generate smaller HDL particles. A related enzyme called endothelial lipase (EL) hydrolyzes HDL phospholipids, generating smaller HDL particles that are catabolized faster. Remodeling of HDL influences the metabolism, function, and plasma concentrations of HDL.

Genomics of Plasma Lipids
Plasma total cholesterol was established as an independent risk factor for coronary heart disease (CHD) through the Framingham Heart Study and others. Subsequently, it was clarified that the two major cholesterol-carrying lipoproteins, LDL and HDL, have opposite associations with CHD: LDL-C is positively associated and HDL-C inversely associated with CHD. Subsequently, plasma levels of TGs were also found to be strongly positively associated with CHD. The study of the genetic determinants of TG, LDL-C, and HDL-C has illuminated the causal relationships of these lipids with CHD. Mendelian conditions in which TG, LDL-C, and/or HDL-C are at the extremes of the population have been extensively studied and have refined our understanding of specific gene products in lipoprotein metabolism (reviewed below). Furthermore, many common and rare variants associated with plasma lipid traits have been identified and used as instruments for Mendelian randomization and tools for understanding physiology (also reviewed below). The genomics of lipid traits is a relatively mature field, and while there remains much to be discovered, lipids are a prime quantitative phenotype for the clinical application of genomic and personalized medicine. Here we update the progress in our understanding of Mendelian disorders of lipoprotein metabolism, and then, we discuss how unbiased genomic approaches have enhanced our understanding of lipoprotein physiology and its relationship to CHD.
Mendelian Disorders of Lipoprotein Metabolism
Studies of the molecular basis of Mendelian disorders of lipoprotein metabolism have provided major insights into the regulation of lipoprotein metabolism, as well as novel targets for therapeutic development ( Table 3.1 ). We first review the Mendelian disorders of high TGs, then high LDL-C, then low LDL-C, and finally extremes of HDL-C.
Mendelian Disorders Causing Elevated Triglyceride Levels
Familial Chylomicronemia Syndrome (FCS)
The familial chylomicronemia syndrome (FCS) is caused by mutations that substantially impair the activity of LPL. The genes in which mutations can cause FCS include LPL , APOC2 , APOA5 , and GPIHBP1 . GPIHBP1 serves to chaperone LPL from its site of synthesis to the endothelial surface and dock it to the endothelium, whereas apoC-II and apoA-V circulate on lipoproteins and both promote the activity of LPL. Loss-of-function mutations in both alleles of any one of these genes can cause FCS, with LPL mutations the most common. Rarely, double heterozygotes with an FCS phenotype have been reported. The prevalence of FCS is not well established.
FCS is characterized by extreme hypertriglyceridemia (often >1000 mg/dL), sometimes associated with physical findings such as eruptive xanthomas, lipemia retinalis, and/or hepatosplenomegaly. Acute pancreatitis is the most important clinical consequence of FCS, and can be recurrent and life threatening. Interestingly, despite the markedly elevated TG (and cholesterol) levels, premature ASCVD is not generally a feature of this disease.
Historically, the primary approach to management has been restriction of total dietary fat. Fibrates and fish oils are often relatively ineffective. However, new approaches to therapy are in clinical development, including targeting APOC3 with an antisense oligonucleotide and ANGPTL3 with an antibody . Therefore, there is increasing interest in making the molecular diagnosis of FCS through a targeted gene sequencing approach. If the new therapies are eventually approved, FCS is likely to become a classic candidate for the application of genomic and personalized medicine, with genomic diagnosis followed by a personalized therapeutic approach.
Familial Dysbetalipoproteinemia
Familial dysbetalipoproteinemia (FD), also known as Type III hyperlipoproteinemia, is caused by mutations in APOE . Hepatic uptake of chylomicron and VLDL remnants is mediated by the binding of apoE to receptors in the liver. Mutations in APOE that substantially impair its ability to bind to these receptors cause FD. By far the most common molecular basis of FD is homozygosity for a common variant known as APOE2, which differs from the wild-type APOE3 by a substitution of a cysteine for an arginine at position 158. ApoE2 has impaired binding to lipoprotein receptors such as the LDL receptor, resulting in defective removal of chylomicron and VLDL remnants. About 0.5% of individuals are homozygous for apoE2 but the prevalence of FD is only about 1 in 10,000, indicating that other genetic or environmental factors are required for expression of the phenotype. Uncommonly, other rare mutations in APOE can have a dominant negative effect on binding and cause FD in the heterozygous state.
FD is characterized by substantially elevated plasma levels of both TGs and cholesterol, sometimes accompanied by palmar xanthomata (orange creases in the palms) and/or tuberoeruptive xanthomata on the elbows, knees, or buttocks. Premature ASCVD is a major consequence of this disorder. If suspected clinically, a molecular diagnosis can be made by ordering APOE genotyping; the finding of the homozygous apoE2/E2 genotype in the appropriate clinical setting is diagnostic. (Note that some laboratories will also perform genotyping for APOE4 and report out a positive result, which can be problematic given the strong association of APOE4 with Alzheimer’s disease.) In the event of a strong clinical suspicion and negative APOE2 genotyping, sequencing of APOE for rare dominant mutations can be considered. In addition, HL deficiency, caused by loss-of-function mutations in both alleles of the LIPC gene, can cause elevated remnant particles similar to FD and could be considered in the differential diagnosis.
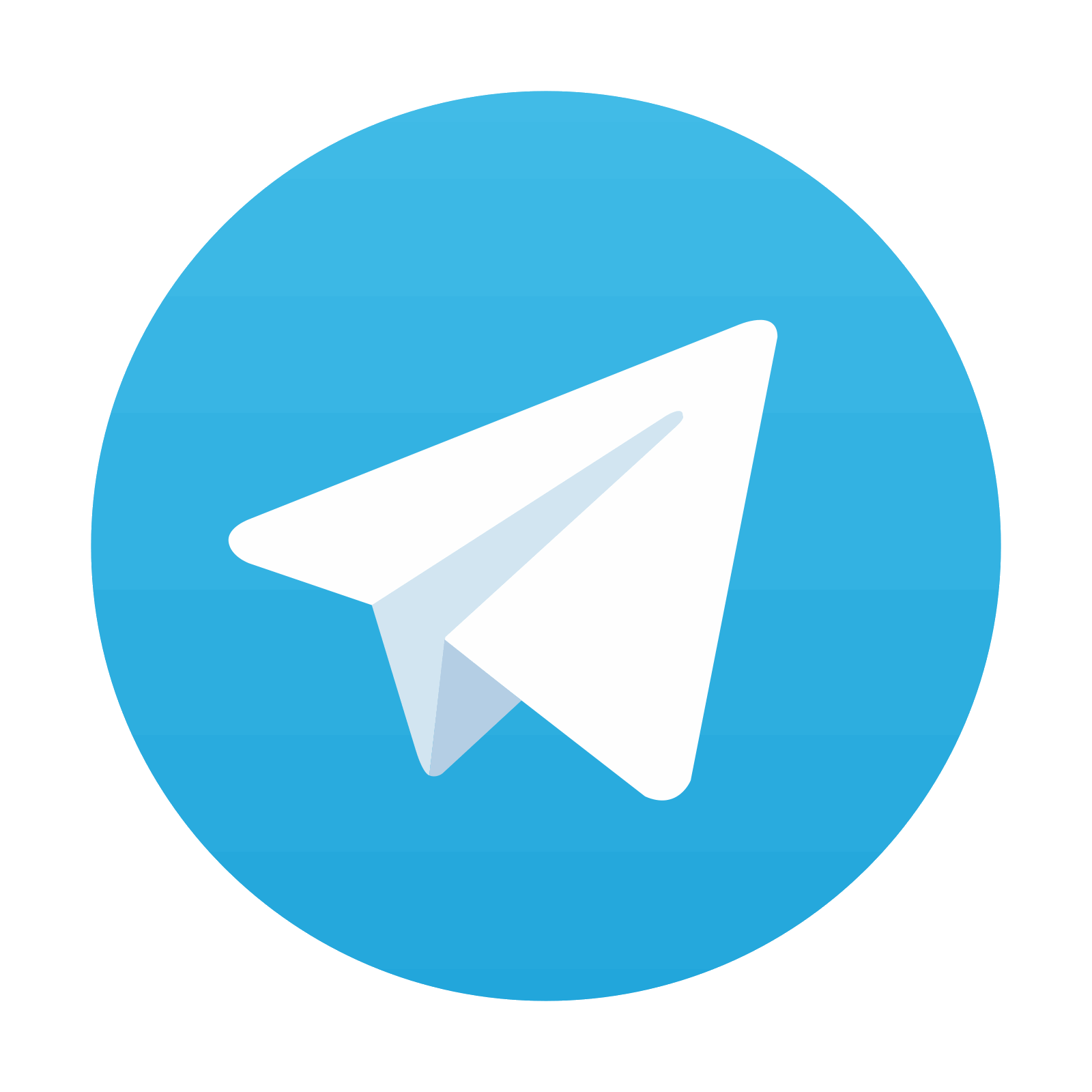
Stay updated, free articles. Join our Telegram channel
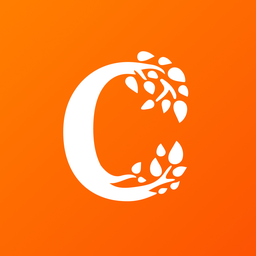
Full access? Get Clinical Tree
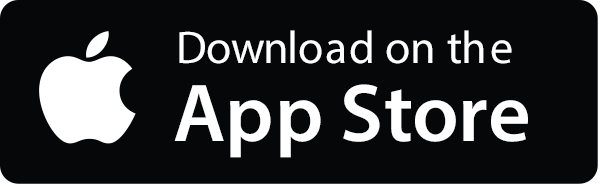
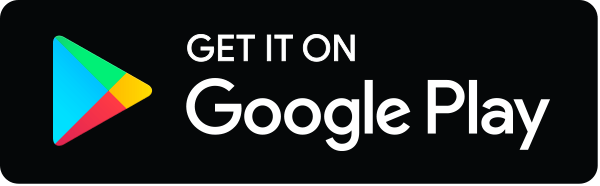