PET instrumentation has undergone several incremental improvements in recent years. This chapter will discuss recent technology advances for PET/CT and PET/MR, which include improved sensitivity, new detectors, digital PET, improved electronics advanced reconstructions, implementation of time-of-flight (TOF), and motion compensation methods. We also review how these high-quality CT component and hybrid PET/MR systems enable some additional potential applications for advanced hybrid cardiac imaging.
Historically, PET scanners operated in 2D mode, with interplane septa between slices and with each slice reconstructed independently. This technique had the advantage of reducing the scatter, randoms, and singles rate of the PET scanner. This improvement in image quality came at the expense of system sensitivity. In 3D PET, there are no interplane septa that physically separate detector rings along the axial dimension, which improves count sensitivity by a factor of 4–6, as compared to traditional 2D PET scanners with interplane septa. However, the disadvantage of 3D acquisitions is an increased scatter fraction, with the number of scattered events detected as a percentage of all coincidence events in the 30%-40% range.1 As the technology for reducing system dead time and scatter correction improved, most instrumentation providers embraced the 3D imaging mode, with many modern PET/CT systems being built without a 2D option.
Importantly for first-pass cardiac imaging, with short-lived tracers such as 82Rb or 15O water, the number of single events is very high, and consequently so is the number of multiple random coincidence events (random events), which are primarily produced by the simultaneous occurrence of a true coincidence event and a single event or two single events in the detectors. This may lead to event pileups and saturation during initial phases of the first-pass cardiac imaging with PET. Clinical PET studies optimize the injected doses of 82Rb for the new 3D PET scanners, thereby avoiding the saturation issues.2 Manufacturers also introduced specially designed high-count rate detectors to withstand the high-count rates during initial phases of dynamic 82Rb imaging. However, challenges still remain with analog PET systems for the high-count rate with 82Rb.3 Despite these potential technical difficulties with 3D PET kinetic imaging, the advantages of lower injected doses and a lower overall radiation burden for the patient make 3D PET protocols preferred.
When performing 3D PET imaging with short-lived tracers such as 82Rb, attention must be paid to the approximately 13% of nuclear decays associated with a 776 keV prompt-gamma emission. These prompt gammas occur when the 82Rb nucleus decays into an unstable final state, which then decays to its ground state releasing the 776 keV photon. These prompt-gamma photons can downscatter into the 511 keV PET energy window. Prompt-gamma corrections have been now incorporated in the 3D reconstruction process together with corrections for standard scattered events. This was not necessary for 2D PET imaging, but is very important for cardiac 3D PET 82Rb imaging with the new generation of scanners.4
An important aspect of the PET system is the choice of the scintillation crystal used for annihilation photon detection. The density of the detector crystal will affect its ability to stop incoming 511 keV radiation completely on the first interaction. The high light yield (expressed as the number of light photons emitted for each MeV of energy absorbed) is desirable to improve signal-to-noise and scatter photon discrimination characteristics. Finally, the speed of the scintillation and the decay of the light impulse after absorbing the annihilation photon will affect PET system count-rate capability, which will be important in first-pass dynamic acquisitions with short-lived tracers and time of flight (TOF) capabilities. A summary of common PET scintillators is given in Table 6-1. Active research is currently underway with the goal to develop superior PET scintillation crystals, which feature high-density and high-atomic numbers, necessary for an efficient photoelectric conversion of the annihilation photons, and short decay time of the scintillation light.5,6
The size of the individual scintillation crystals in a block of the detector ranges typically from 4 × 4 × 20 mm3 to 6.3 × 6.3 × 30 mm3 for the PET/CT scanners listed in Table 6-2. Most traditional PET scanners use analog photomultiplier tubes (PMT), which are much larger than the individual detectors in the detector block to which the tubes are coupled; therefore the interpolation of the signals between neighboring PMTs (Anger principle) is needed to determine the γ-ray’s original impact. Because of this, the use of analog PMTs limits possible improvement of spatial resolution in PET imaging, by the use of smaller crystals.
Model | Ingenuity TF | Vereos | Discovery IQ (5-ring) | Discovery MI (4-ring) | Biograph mCT | Biograph Vision 600 | Signa PET/MR | Biograph mMR |
---|---|---|---|---|---|---|---|---|
Patient port [cm] | 70 | 70 | 70 | 70 | 78 | 78 | 60 | 60 |
CT [slices] | 64-128 | 64-128 | 16 | 32-128 | 20-128 | 64-128 | N/A | N/A |
Maximum patient weight [kg (lbs)] | 195 (430) | 195 (430) | 226 (500) | 226 (500) | 226 (500) | 226 (500) | 226 (500) | 200 (441) |
Plane spacing [mm] | 2 or 4 | 1, 2, or 4 | 3.3 | 2.8 | 2 | 1.6 | 2.8 | 2 |
Crystal size [mm3] | 4.0 x 4.0 x 22 | 4.0 x 4.0 x 22 | 6.3 x 6.3 x 30 | 3.95 x 5.3 x 25 | 4.0 x 4.0 x 20 | 3.2 x 3.2 x 20 | 4.0 x 5.3 x 25 | 4.0 x 4.0 x 20 |
Number of crystals | 28,336 | 23,040 | 19,200 | 19,584 | 32,448 | 60,800 | 20,160 | 28,672 |
PMTs | Analog | Digital SiPM | Analog | SiPM | Analog | SiPM | SiPM | APD |
Physical axial FOV [cm] | 18 | 16.3 | 26 | 20 | 21.8 | 26 | 25 | 25.8 |
Detector material | LYSO | LYSO | BGO | LxSO** | LSO | LSO | LxSO** | LSO |
System sensitivity % | 0.74 | 0.57* | 2.2 | 1.35 | 0.95 | 1.6 | 2.1 | 1.5 |
Transaxial resolution @ 1 cm [mm] | 4.7 | 4.0 | 4.9 | 4.5 | 4.4 | 3.2 | 4.2 | 4.1 |
Transaxial resolution @ 10 cm [mm] | 5.2 | 4.5 | 5.5 | 4.5 | 4.9 | 3.8 | 5.2 | 5.2 |
Axial resolution @ 1 cm, [mm] | 4.7 | 4.0 | 5.1 | 4.8 | 4.5 | 3.4 | 5.8 | 4.3 |
Axial resolution @ 10 cm, [mm] | 5.2 | 4.5 | 5.5 | 4.7 | 5.9 | 3.7 | 7.1 | 6.6 |
Peak NECR [kcps] kBq/@ ml* | 120 @ 19 | 171@50*** | 120 @ 9 | 180 @ 20 | 175 @ 28 | 300@30 | 210 @ 17.5 | 175 @ 21.8 |
Time-of-Flight resolution [picosecs] | 550 | 345 | N/A | 385 | 540 | 214 | 400 | N/A |
Time-of-Flight localization [cm] | 8.9 | 5.2 | N/A | 5.8 | 8.1 | 3.2 | 6.0 | N/A |
Coincidence window [nanosecs] | 4.5 | 1.5 | 9.5 | 4.9 | 4.1 | 4.7 | 4.6 | 5.9 |
Digital imaging systems replace the analog photomultiplier tubes with silicon digital photomultipliers (SiPMs),7 blocks of avalanche photodiodes (APCs),8 or analog SiPMs.9 More compact size of digital detectors allows to achieve one-to-one coupling of the between digital photomultipliers and scintillation crystal blocks (Table 6-2, Figure 6-1). In addition, these digital detectors are not affected by magnetic fields, making them particularly useful in PET/MR systems.
SiPMs are built from APCs and will detect light events in serially connected APCs. The total gain in the light signal in the SiPM is similar to that obtained in the analog PMT (approximately 106). One design used in digital PET connects multiple SiPM pixels together and adds the signals from each pixel for a summed output, which requires so-called application-specific integrated circuits (ASICs) to condition the signal and minimize the interference from the electronic noise or unstable baseline currents (present without any counts). This type of design is sometimes referred to as the analog SiPM. Analog SiPM tends to consume more power and generate more heat than digital SiPM.10 True digital SiPM incorporates electronics to each photodiode to achieve a single readout for each photodiode. The digital SiPM design has been used in the preclinical system designed as a PET insert for the standalone MRI scanner11 and in the Philips Vereos system.12
Both digital and analog SiPMs have very good intrinsic timing resolution, and are therefore well suited for use in conjunction with TOF PET acquisition (described further in the chapter), potentially achieving simultaneous improvements in sensitivity, image resolution, and maximal count rate. Digital counting can potentially improve reading the digital signals from the detectors at the high-count rates. The maximal count rate is very important for the myocardial flow measurements with short-lived PET tracers, such as 82Rb.13 SiPMs in the clinical system can match crystal size, currently as low as 4 mm (Table 6-2). However, SiPM or avalanche photodiodes can be fabricated easily in even smaller sizes and could be matched in the future with much smaller size crystals, if required. In comparison, the size of conventional analog PMT will limit the accuracy of photon positioning detection for crystal element sizes of less than 4 × 4 mm. It is likely that the imaging resolution of digital PET is improved further with the use of smaller detector elements. In cardiovascular imaging, this is particularly important for vascular PET applications, device infection, and sarcoid imaging where high image resolution is of utmost importance. Examples of new digital PET detectors are shown in Figure 6-1.
All current digital PET designs still require scintillation crystals for the detection of the annihilation photons, because the digital detectors detect the scintillation photon rather than the annihilation photons directly. It is possible that in the future, fully digital PET detectors without photon conversion to visible light will provide even greater image quality benefits for PET.14 However, such designs are currently hampered by the poor timing performance of CdTe and CZnTe solid state radiation detectors, which is currently about 10 ns (compared to the 0.3 ns of current state-of-the-art PET systems). Some novel solutions to this limitation for CdTe solid state detectors have been recently proposed.15,16 However, none of the current clinical systems or clinical prototypes to date are using such direct radiation detection solid-state technology.
Typical axial coverage of PET in one bed position varies from 16 to 26 cm. The smallest 16 cm axial coverage is sufficient for the typical cardiac imaging protocols. Larger axial coverage may be sometimes required to include aorta in the field of view (FOV) for heart imaging, and for carotid imaging. The larger axial coverage is achieved in the hardware by extending more PET detector rings (so called 4-ring and 5-ring systems). These systems are primarily designed for oncology applications, since the extended axial coverage allows reduced number of bed positions for whole-body imaging and consequently faster overall imaging time. However, there is a trade-off: the longer axial FOV requires more detectors and more crystals, increasing the cost.
For cardiac applications, there are no obvious benefits of extended bore length since the standard coverage for the typical 3-ring system of about 16 cm in one bed position is typically sufficient to cover the heart region. In addition, there could be potentially negative consequences of longer bore in a PET scanner for cardiac imaging. Since the scatter fraction during PET imaging is typically increased with the longer bore, the effects such as gamma-prompt with 82Rb imaging are dramatically increased. This limitation would not be present if an 18F agent such as FDG or F-18-flurpiridaz were used. If not appropriately corrected, this may lead to significant artifacts, mimicking myocardial perfusion defects. In addition, extended coverage of the PET scanner may require acquisition of larger number of slices for the CT attenuation correction (AC) map, even if they do not cover the heart. This is because it is usually not possible to perform AC with a reduced FOV using the clinical software, since iterative 3D scatter correction algorithms require a match between the CT and PET axial coverage for accurate results. Thus, purely for cardiac applications, the shorter axial coverage of the PET scanner may be preferable.
All current PET/CT systems use iterative PET reconstruction. New reconstruction methods offer great potential for improved noise statistics17 and improved image fidelity.18 Ordered subset expectation maximization (3D-OSEM) has been implemented on all systems to reduce reconstruction time of iterative reconstruction algorithms.19 Despite OSEM acceleration, computational time can still pose significant limitations, when using standard workstations, particularly for cardiac PET imaging utilizing dynamic and gated protocols. In a typical cardiac protocol, it is possible to have up to 20–30 dynamic and 8-bin gated stress/rest acquisitions in addition to the static stress/rest perfusion images. Thus, the reconstruction time for typical cardiac protocol can be in the order of two magnitudes longer than in standard static imaging. However, it has been shown that iterative techniques yield improved image accuracy and less noise, compared to filtered backprojection for kinetic myocardial flow imaging,20 and, therefore, these techniques are recommended despite longer processing times, even for kinetic imaging with multiple dynamic frames.
During iterative reconstruction, various processes can be modeled, including photon attenuation, scatter, random events, and various resolution degrading defects. One of the most important corrections recently introduced by all vendors is point spread function (PSF) modeling, which corrects for various resolution-degrading effects in the PET system. The knowledge of the variation of the system PSF across the FOV is required for this correction. This could be obtained by recording a 3D map of measurements of moving point sources across the entire 3D FOV of the scanner.21 The measured 3D maps of PSF responses can be stored for each specific PET scanner model. The PSF resolution in the PET scanner typically deteriorates towards the edge of the FOV. The PSF corrections can be built into the iterative reconstruction process. This method has been implemented on Siemens as the High-Definition option22 and on GE as the SharpIR option23 (Figure 6-2). On the Philips PET system, PSF modeling is implemented postreconstruction as a separate iterative computation using the Richardson-Lucy algorithm, designed previously for applications in astronomy.24 Similar to Siemens HD, the implementation of this technique also requires the estimation of the PSF 3D maps scanner in the FOV.
PSF correction or resolution recovery can dramatically increase the values of standardized uptake value (SUV) measurement of a lesion when the size of the lesion is smaller than the resolution of the imaging system. Effective tomographic image resolution as low as 2 mm (in the air) and significant improvements in image contrast have been achieved using resolution recovery,25 and better definition of subtle myocardial perfusion defects have been reported in clinical settings with the use of this approach.26
In coronary PET studies with small lesions, the use of PSF correction is important to restore the signal and improve the signal-to-noise ratio (SNR) resulting in a signal increase of up to 4 times in small coronary lesions,27 as seen in Figure 6-3. The use of PSF has also been studied for dynamic myocardial flow studies to reduce low-statistics bias in quantitative dynamic PET.28 The effect of the new reconstruction techniques with PSF on dynamic PET imaging is discussed in detail in a comprehensive technical review.29
Figure 6-3
Coronary Phantom Study of Resolution Resolution Recovery With Point Spread Function (PSF). Static phantom acquisition of simulated cylindrical coronary lesion (arrows): 2 mm diameter, volume 44 mm3, 6 mm from myocardium on a Siemens scanner with HD reconstruction (resolution recovery). The lesion/myocardium 18F concentration was 4:1. In this example, contrast improved 4 times with HD resolution recovery reconstruction.

Typically, the use of iterative reconstruction requires postfiltering to reduce image noise. This is usually accomplished by 3D Gaussian smoothing with kernels, such as 4 or 5 mm, to match the desired imaging resolution. An advancement in the techniques for noise suppression recently implemented by some (but not all) vendors is noise regularization during iterative reconstruction by statistical modeling. One example is Bayesian penalized likelihood, which utilizes additional constraints and knowledge about the image data to suppress noise while preserving details. Bayesian penalized likelihood regularization is implemented in the Q.Clear reconstruction method from GE.30 This relatively new approach has not yet been used widely in cardiovascular imaging and is not available for all scanners. In preliminary studies, it has been reported that Bayesian penalized likelihood techniques result in less noise than comparable reconstructions obtained with filtered back projection (FBP) and OSEM, and do not cause bias in the estimation of the input function, during cardiac dynamic imaging.31 Further work is required to validate these advanced techniques for various cardiovascular PET protocols.
One of the notable improvements in the next-generation PET scanners is the use of TOF information, achieved by the fast decay time of the new crystals (LSO and LYSO) combined with fast coincidence electronics. The principle of TOF PET was investigated and proposed early in PET development,32 but until recently has not been implemented in clinical PET scanners.
The TOF principle is based on estimating the time difference between two events of annihilation photons hitting the crystal at opposite sides of the detector ring (Figure 6-4). If Δt is the difference in the detection times of two photons originating from the same positron annihilation and c is the velocity of light (3 × 108 m/sec), the positron annihilation location along the path (called line of response) of two photons with the reference to the center of the detector can be estimated as P = Δt × c/2.
Figure 6-4
Principle of Time-of-Flight Imaging in PET. Rectangles represent detector crystals. For standard PET, coincidence event detected in A and B define a line along which the origin of the event is located. For time-of-flight PET, the difference in the time of arrival is measured and the location of the origin is assumed to be located in some section of the line joining detectors C and D. Shaded pixels represent possible pixels that contain the origin of the event. Note that for the TOF, the number of these pixels is substantially smaller indicating more precise localization. (Reproduced with permission from Sitek A: Data analysis in emission tomography using emission-count posteriors, Phys Med Biol. 2012 Nov 7;57(21):6779-6795)

The accuracy of the estimation of the positron annihilation position is related to the timing resolution of the detector and front-end electronics. The TOF localization accuracy of current TOF clinical PET system ranges from 5.2 to 8.2 cm corresponding to the TOF timing resolution of 345–544 picoseconds (Table 6-2). This estimation of the location of the event along the line of response can be then used in the reconstruction algorithm to reduce noise and scatter, resulting in higher SNR in the TOF-reconstructed images. It has been demonstrated that for large objects, the gain of the SNR with TOF, as compared to images reconstructed without TOF information, can be approximated by the g = √(D/a) where D is the detector field of view (usually 60 to 80 cm) and a is TOF positioning accuracy.33 From Table 6-2, we can see that typical TOF SNR gains could be approximately in the 3-4 range.
Vendors have used this estimated SNR gain attributed to TOF to provide an effective net equivalent count rate (NECR) and effective system sensitivity, by simply multiplying the non-TOF baseline NECR or sensitivity by the TOF gain. This can lead to confusion when comparing the characteristics of TOF and non-TOF PET scanners. Because of vendor differences in measuring TOF NECR numbers, direct comparison of these values is not possible (Table 6-2). Furthermore, the absolute sensitivity of the TOF scanner (percent of detected events) is not changed as compared to the non-TOF scanner. Similarly, TOF gain will not guard against potential system saturation with 82Rb scanner, and therefore raw NECR number should be considered for these applications.
The strongest clinical advantage of TOF seen when it is used in combination with PSF correction.34,35 However, the effects TOF and PSF in cardiac imaging differ significantly between vendors36 because their implementations of these corrections are unique and proprietary. It has been recently shown that TOF imaging results in contrast improvement and increased uptake in the lateral wall for N-13 ammonia myocardial perfusion PET imaging37(Figure 6-5). Estimation of TOF SNR gains for 18-FDG cardiac viability patients has been reported recently.38 The average overall clinical SNR increases were in the order of 21% when TOF was used. Clinically, these gains may be of most importance in obese patients, where SNR is low.39 For cardiac 82Rb myocardial blood flow imaging, it has been demonstrated that the use of TOF can lead to more consistent reconstruction results.40 A note of caution is needed for dynamic blood flow quantification with 82Rb and TOF reconstruction. A recent study found a considerable increase in the flow values when TOF was used, as compared to standard iterative reconstruction.41 TOF techniques have been primarily developed for different tracers and 82Rb could require special considerations because of longer positron range and prompt-gamma corrections.4 TOF imaging may be of particular importance for the high-resolution PET imaging vascular imaging or imaging with high-resolution myocardial perfusion tracers, such as 18F-flurpiridaz. Advanced coronary PET imaging with 18NaF has been reported with TOF system42–45; however, to date it has not yet been compared to non-TOF imaging.
Figure 6-5
Comparison of Images. The comparison of images is between standard 3D iterative reconstruction (top) and TOF reconstruction (bottom) for N-13 ammonia myocardial perfusion PET imaging in a case with three-vessel coronary artery disease. There is a severe decrease of uptake in the inferior wall more pronounced with TOF. (Reproduced with permissions from Tomiyama T, Ishihara K, Suda M, et al: Impact of time-of-flight on qualitative and quantitative analyses of myocardial perfusion PET studies using (13)N-ammonia, J Nucl Cardiol. 2015 Oct;22(5):998-1007)

AC for PET is mandatory owing to the need to record both the forward traveling and backward traveling photons to record a true coincidence event. The result is that the total attenuation of a true event is the total attenuation along the line of site (as opposed to SPECT where the attenuation begins where the nucleus decay, inside the patient and continues to the detector face). AC for PET was originally implemented with radionuclide transmission rod-sources, which were used to create patient photon attenuation maps. However, all modern commercial systems now use CT-based AC or MR-based -AC. High-quality CT-based attenuation maps can be obtained within few seconds on current PET/CT scanners.46 There are significant quantitative PET differences between traditional AC by transmission sources with 68Ge or 137Cs sources and CT-based AC. These differences may remain even after alignment of the CT maps to the emission data, particularly when there are metal-induced artifacts caused by, for example, pacemakers or implantable cardioverter defibrillators in the CT images.47,48 Vendors employ various software algorithms for the correction of metal artifacts in the CT scans.49 When these methods are applied to the CT attenuation maps, they result in increased quantitative accuracy of PET.
When CT maps are used for the AC, one issue is the potential misalignment between CT and PET images caused by respiratory or patient motion,50 a particularly important factor in imaging of the heart.47,51 It has been demonstrated that PET/CT AC map misalignment can affect the results in about 40 to 60% of myocardial perfusion imaging.47,52,53 Careful visual verification of the alignment is needed to minimize misregistration artifacts. Automatic correction of the misalignment registration of CT and PET maps to reduce these artifacts have been developed by vendors.54 In a recent study, it has been demonstrated that the automatic alignment of the CT-based AC maps is superior to the clinically performed manual quality control (QC) and alignment by the PET technologists.53 Figure 6-6 shows an example of potential misdiagnosis caused by misregistration in the cardiac 82Rb study.
Figure 6-6
False-Positive Nuclear Scan. The false-positive result is caused by the CT attenuation correction (CTAC) scan registration error resolved by the automatic correction in a 44-year-old male presenting with shortness of breath. PET and CTAC images fused before alignment (A) and after rigid alignment (B). Misalignment is indicated by the white arrow head (A). Stress perfusion PET images before alignment (C) and after alignment (D) and polar maps are shown with the white arrow indicating the perfusion artifact caused by misalignment. Quantitative plese delete mask green text on figure was 11% (C right), when no correction for alignment was performed (ITPD after manual verification was also 11%) compared to a normal scan (ITPD 1.8%) after automatic registration (D right). Invasive coronary angiography did not show significant coronary artery disease. (Reproduced with permission from Slomka PJ, Diaz-Zamudio M, Dey D, et al: Automatic registration of misaligned CT attenuation correction maps in Rb-82 PET/CT improves detection of angiographically significant coronary artery disease, J Nucl Cardiol. 2015 Dec;22(6):1285-1295)
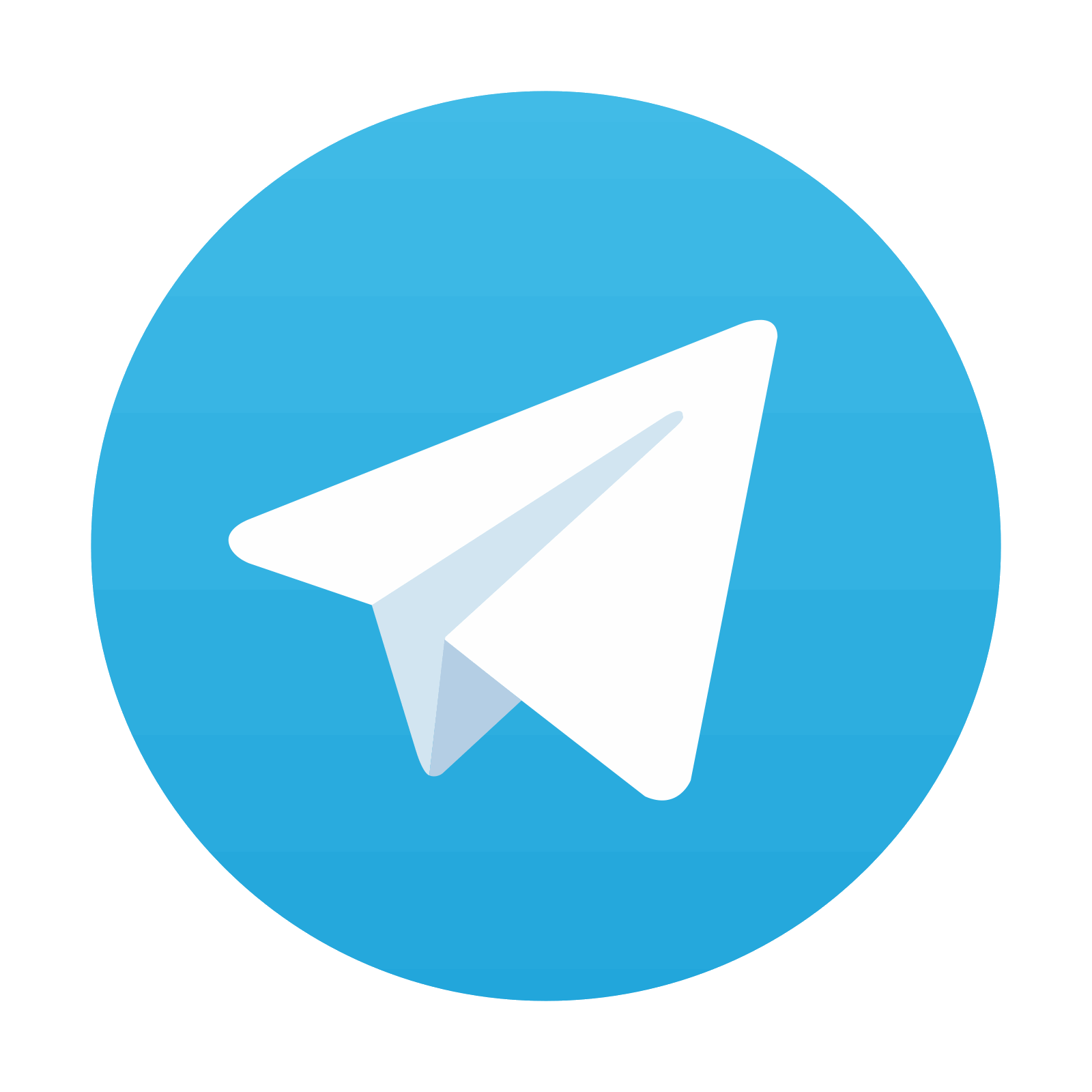
Stay updated, free articles. Join our Telegram channel
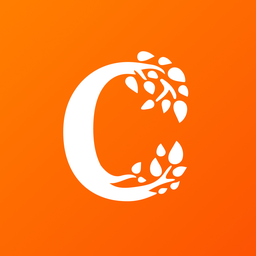
Full access? Get Clinical Tree
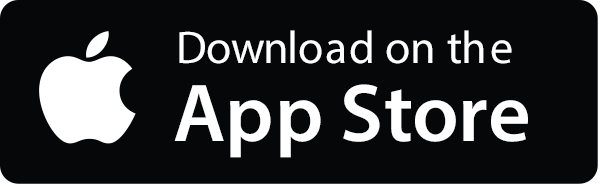
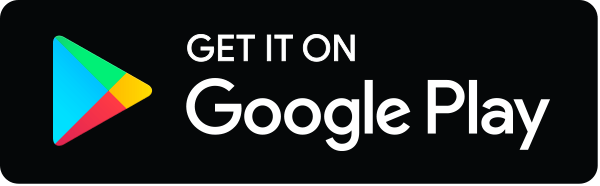
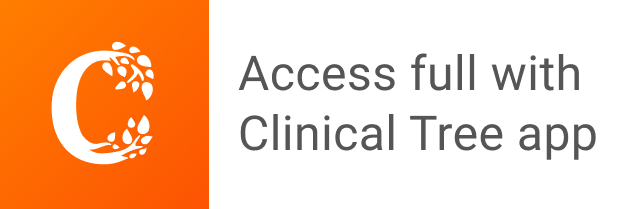