Ischemic heart disease (IHD)
Myocardial mechanics in IHD
Regional function and IHD
In 1935, Tennant and Wiggers demonstrated that a coronary occlusion almost immediately induced a regional wall motion abnormality. Nowadays, such ischemic wall motion abnormalities can be detected by standard echocardiography before electrocardiogram (ECG) changes or angina pectoris occur (the so-called ischemic cascade) and allow an assessment of the localization and extent of the ongoing ischemia.
Stunning and hibernation describe a dysfunction of viable myocardium, which is not proportional to the current blood flow but the result of a previous ischemic event or a longer period of repetitive ischemia. Echocardiography will also detect this regional dysfunction at rest, while a (transient) improvement can be observed under an inotropic challenge with dobutamine.
Scar causes myocardial dysfunction due to the loss of contractile capabilities and altered passive tissue properties when infarcted myocardium has been replaced by connective tissue. Echocardiographic images may show a thin and echogenic wall, potentially an abnormal contour of the ventricle, and a lack of thickening or even systolic bulging in the damaged region.
Conventional assessment of regional function
Regional myocardial function is commonly evaluated by echocardiography based on the visual assessment of thickening and motion of the left ventricular (LV) wall. For this, the wall is subdivided into 16 to 18 segments ( Fig. 8.1 ). The 16-segment model with 4 segments in the apex and 6 segments in the mid and basal part of the ventricle represents the classical recommendation of the American Society of Echocardiography (ASE). It divides the LV wall into regions of approximately similar myocardial volume (see Fig. 8.1 A). A 17th apical segment has been added later to increase the compatibility with nuclear imaging, cardiac computed tomography (CT), and magnetic resonance imaging (MRI) (see Fig. 8.1 B). Quantitative echocardiographic methods such as two-dimensional (2D) speckle tracking are commonly applied to the three apical views separately so that the division of the left ventricle into 6 segments per view is most practical. This results in six apical segments, and with this an 18-segment model of the left ventricle (see Fig. 8.1 C).

In standard echocardiography, segmental function is evaluated in a semiquantitative way, based on the myocardial motion pattern, in which “normokinesia” describes a wall thickening and endocardial inward motion, “hypokinesia” a reduced thickening and inward motion, “akinesia” the loss of thickening, and “dyskinesia” a thinning and outward motion during systole. This evaluation focuses on the radial effects of myocardial contraction, as the motion of the strong contrast between echogenic myocardium and black cavity provides a good target for the eye. The longitudinal function component—although of high diagnostic value—is commonly not considered because it requires the visual interpretation of intramyocardial speckle motion, which is challenging even for a trained reader.
Training and experience are key to a reliable visual interpretation of regional function, and a learning curve exists during which the novice should read echocardiograms only under the supervision of an experienced colleague ( Fig. 8.2 ). Despite this, regional function assessment is subject to interobserver variability, which strongly depends on image quality as well as the severity and extent of the regional abnormality ( Fig. 8.3 ).


Available methods to quantify regional function
Several methods have been proposed to improve the recognition of regional wall motion abnormalities and to facilitate their quantification.
An early method was based on endocardial border detection, color coding the area that the endocardial border had passed in a certain period of time ( Fig. 8.4 ). This “color kinesis” method could only consider endocardial motion relative to the transducer, so that overall heart motion would influence the result. Further, it delivered only an end-systolic snapshot that did not allow comprehensive analysis of subtle changes of timing of contraction.

Yoshida described in 1965 that myocardial motion could be recognized in spectral Doppler signals. It took until the introduction of color Doppler in the late 1980s for this approach to become feasible for diagnostic purposes. The tissue Doppler method is still a useful tool for the evaluation of global properties of the ventricle and plays a central role in the assessment of diastolic function. It has a big limitation for the assessment of regional function because segmental velocities show a base-to-apex gradient that prevents the use of uniform cutoff values for the detection of regional dysfunction ( Fig. 8.5 A). Furthermore, adjacent segments interact (tethering) so that localization of dysfunctional regions based on abnormal velocity patterns is very difficult.

With the introduction of tissue Doppler–based strain imaging in the late 1990s, regional myocardial deformation became measurable independent from overall heart motion and the functional state of adjacent myocardium (see Fig. 8.5 B). Investigators focused mainly on longitudinal function because it is the only deformation component assessable in all LV segments. The tissue Doppler method still offers the best temporal resolution of all noninvasive cardiac imaging modalities and is relatively robust in the presence of moderate image quality. Nevertheless, its application is cumbersome when aiming at a comprehensive assessment of the entire ventricle, and it requires experience in the handling of artifacts, which prevents a broader use in the clinic.
Advances in computer technology allowed the application of feature tracking in moving cardiac images so that 2D speckle tracking became commercially available in the early 2000s. It is easy to use, is widely automated, and provides complementary clinical information on global and regional function, which makes it currently the standard approach for quantitative regional function assessment.
Regional function assessment by speckle-tracking strain
Tracking features in echocardiographic images provides a noisy motion vector field that requires advanced postprocessing. Noise needs to be smoothed out, and dropouts or disturbances from stationary reverberation artifacts need to be detected and replaced by information from the surrounding tissue. For this, a priori knowledge on the general shape and normal deformation of the heart is implied in the postprocessing software—in other words, a certain continuity in the behavior of adjacent myocardial regions is assumed. Further, as the heartbeat is a cyclic process, it is assumed that myocardium returns to the same shape after one cardiac cycle, and strain curves are consequently forced to zero at each R-trigger point. In this sense, strain data based on speckle tracking are not of better quality than those based on tissue Doppler, but more postprocessing is applied before they appear on display.
Smoothing and postprocessing toward a normal, “expected” behavior of the myocardium bears the risk that true regional abnormalities are missed because smoothing reduces the fidelity in following regional myocardial motion and with this the ability for detecting small regions of dysfunction. On the other hand, smoothing helps to reduce noise and improves inter- and intraobserver reproducibility. Finding the right balance of postprocessing parameters is therefore one of the most challenging tasks of software developers and determines the quality and performance of a speckle-tracking software product. The recent comparison of postprocessing software from different vendors in the context of the joint task force on strain standardization of the ASE and European Association of Cardiovascular Imaging (EACVI) showed several important consequences, including the percentage of segments excluded due to bad tracking ( Fig. 8.6 ), differences in test–retest variability ( Fig. 8.7 ), and differences in measured values ( Fig. 8.8 ). These differences translate to relevant discrepancies in the detection of regional function ( Fig. 8.9 ).




Strain as a marker of inhomogeneous contractility
IHD causes regional inhomogeneity of myocardial contractility. Myocardial segments with normal and abnormal function interact, and as a result regional strain curve patterns may substantially deviate from the normal shape. This requires particular care when measuring strain. Whereas global strain can be described by measuring its peak value, which always occurs around end systole, describing regional deformation requires at least the distinction of end-systolic strain (i.e., at aortic valve closure) and a potential postsystolic peak (usually during isovolumetric relaxation or very early in diastole). Besides that, lengthening during systole might occur ( Fig. 8.10 ). Therefore three strain values (systolic strain, end-systolic strain, postsystolic strain) and one index (postsystolic index) can be distinguished, and attempts to use a single parameter are likely to prove inferior to assessing the shape of the curve.

Timing of strain measurements
Given the previous information, correct assessment of regional dysfunction requires reliable timing. Most speckle-tracking software packages use an ECG R-wave trigger for defining the zero reference of the strain curve. Although this is a good surrogate for end diastole in normal QRS morphology, distorted QRS complexes (e.g., in the presence of conduction delays) may result in a late trigger signal and thus a wrong definition of the zero reference of the strain curve ( Fig. 8.11 ).

Many software packages use the global strain or LV volume curve nadir for defining end systole. This is mostly a good surrogate, but in views that contain several segments with delayed shortening peaks (e.g., due to a large infarct zone), the global nadir does not coincide any longer with aortic valve closure ( Fig. 8.12 ).

The best option is for the definition of end diastole and end systole to be adjusted manually. Information about the true mitral and aortic valve closure time can be most easily derived from the observation of the valves in an apical long axis view. Therefore, some tracking software solutions suggest the user to start the analysis with this view to get the time correct from the beginning. Timing information can also be imported from measurements on spectral Doppler signals of the two valves. The common time base for synchronizing the different acquisitions is the ECG trigger. Consequently, care should be taken that the heart rate variability is limited (<10%) when using this approach.
Software that does not allow a manual timing correction cannot provide exact measurements in regional dysfunction and should be avoided.
Postsystolic shortening
Within seconds, acute ischemia leads to delayed and reduced systolic shortening combined with postsystolic shortening after aortic valve closure. This postsystolic shortening can be understood as a sign of delayed relaxation—effectively, the ischemic region is shortening while LV pressure drops and the surrounding tissue is already relaxing. With longer ischemia, the ischemic region stretches during systole, and the following postsystolic shortening may be rather a sign of passive recoil than delayed relaxation. The functional changes are restricted to the area at risk.
Postsystolic shortening per se is a sensitive yet unspecific marker for regional dysfunction and can also be found in chronic ischemic scar as well as other pathology with regional scar formation, such as localized hypertrophic cardiomyopathy or Fabry disease.
Minor postsystolic shortening with normal systolic function is a frequent finding in 30% to 40% of the myocardial segments of healthy hearts. It occurs predominantly in the apical and basal segments of the inferior septal and anteroseptal walls. Such minor postsystolic shortening in normal segments always follows a normal systolic shortening, so that its relative magnitude rarely exceeds 20% of the total strain curve amplitude. Pathologic postsystolic shortening is usually preceded by systolic stretching of variable length in the beginning of systole and a reduced or no shortening. Pathologic postsystolic shortening accounts for >20% of the total strain curve amplitude ( Fig. 8.13 ).

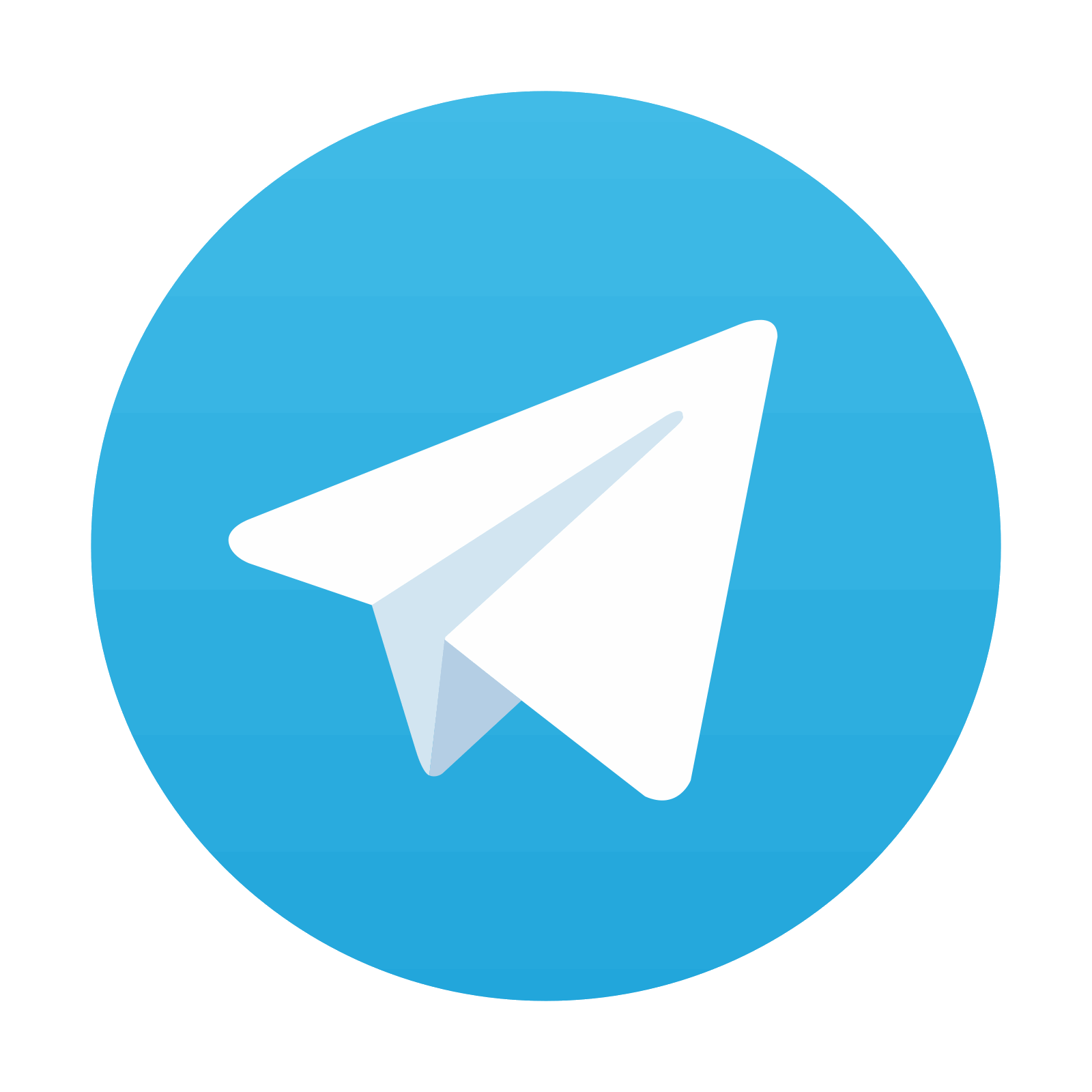
Stay updated, free articles. Join our Telegram channel
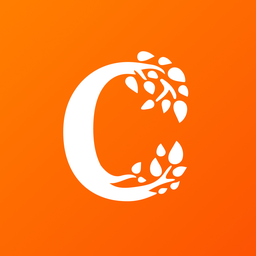
Full access? Get Clinical Tree
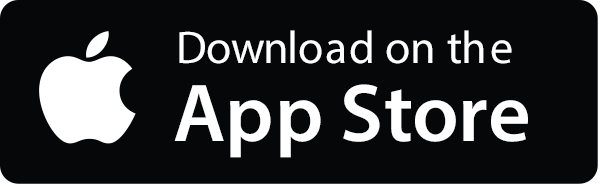
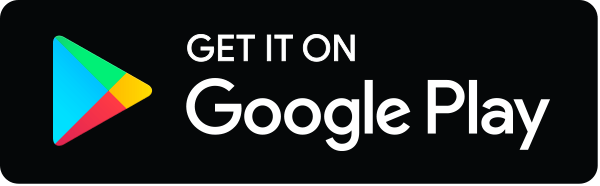
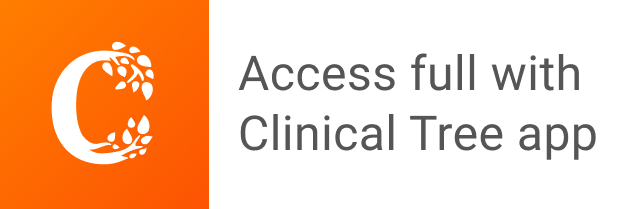