Fig. 8.1
Survival of patients with ischemic cardiomyopathy compared to those with a non-ischemic etiology. Left panel: Patients with ischemic cardiomyopathy have a higher mortality risk than do those with non-ischemic cardiomyopathy. Right panel: The mortality risk increases with more widespread coronary artery disease. Source: Duke Database, Felker et al. J Am Coll Cardiol. 2002;39(2):210–8 [3]
The diagnosis of ischemic cardiomyopathy is straightforward in patients with extensive prior myocardial infarction (particularly involving the large perfusion field of the left anterior descending artery) and stenosis of all three coronary arteries or the left main coronary. However, it can be difficult to differentiate ischemic from non-ischemic etiologies in many patients because of the ubiquitous nature of coronary atherosclerosis in older patients [5]. Heart failure in patients with limited coronary disease (e.g., single-vessel disease) should prompt a search for other causes of cardiomyopathy. In addition, patients can have more than one etiology of heart failure, particularly synergistic combinations such as combined valvular disease and coronary artery disease.
Mechanisms of Ischemic Cardiomyopathy
Normal Control of the Myocardial Blood Flow
Myocardial blood flow at rest is about 0.8 ml/g muscle/min and can rise to about 2.5–3.0 mL/g/min with increased myocardial oxygen demand [6]. Blood flow is autoregulated normally by dilation and constriction of the coronary arterial tree [7]. The delivery of oxygen is tightly coupled to the cardiomyocyte needs. Increased demand caused by increases in myocardial workload lead to coronary dilation and increased conductance of blood (and oxygen). Similarly, reductions in blood oxygen-carrying capacity (usually due to anemia) lead to reduction of coronary resistance to increase coronary blood flow and maintain oxygen delivery.
The coronary tree can be divided arbitrarily into four zones. The large arteries that can be seen on an angiogram or with the naked eye are more than 400 μm in diameter (◘ Fig. 8.2). These arteries are often referred to as conduit arteries because they normally provide very little resistance to blood flow. For example, the blood pressure in the left anterior descending artery when it reaches the cardiac apex is about 95 % of the blood pressure in the left main coronary .


Fig. 8.2
A schematic of the coronary circulation . The large epicardial coronary arteries are conduits to the microcirculation and provide little resistance. The vessels close to the capillary bed dilate and constrict in response to metabolic needs of the cardiomyocyte to match oxygen demand and delivery. The larger microcirculation dilates primarily through endothelial mechanisms
The large conduit arteries divide into the proximal microcirculation (vessels 200–400 μm in diameter). The smooth muscle of these vessels actively constricts and dilates in response to luminal blood flow velocity, autonomic tone, and circulating vasoactive compounds such as catecholamines, vasopressin, and serotonin.
The distal microcirculation (vessels with a thin, smooth muscle media and luminal endothelial layer) extends from the proximal microcirculation to the capillary level. These very small arteries are bathed in the extracellular fluid of cardiomyocytes. They respond by dilating and constricting to mediators of oxygen need (such as pH and adenosine) produced by the cardiomyocytes. This response links demand with oxygen delivery.
The tone and caliber of arteries from the coronary ostium to the microcirculation (i.e., vessels 200 μm diameter or larger) are modulated by endothelial production of dilators (primarily nitric oxide, NO). In their native state, the coronary arteries are prone to constrict were it not for the continual generation of NO by the enzyme NO synthase within the endothelial cell (eNOS). With increases in myocardial metabolic oxygen requirements—such as increase in heart rate, blood pressure, or preload—the microcirculation closest to the myocyte (microcirculation of 50–200 μm) dilates to increase blood flow.
In most mammals , including humans, the artery seeks to maintain a flow velocity of 12–17 cm/s. When the distal microcirculation dilates, the velocity of flow increases in the upstream coronary, stimulating shear stress receptors on the luminal side of the endothelial cell. This causes the endothelium to release more NO. The NO permeates the underlying vascular smooth muscle, leading to an “ascending wave” of coronary dilation.
This combination of distal microcirculation dilation in response to the myocyte need and the ascending dilation caused by NO release reduces coronary resistance and increases myocardial blood flow. In this way, the blood flow to the myocardium is closely paired to the metabolic requirements. This coupling of blood flow to myocardial metabolic need enables the cardiomyocyte to rapidly increase contractility and shortening.
Coronary Atherosclerosis
The most common cause of IschCM is atherosclerosis of the large coronary arteries. About six million people in the USA suffer from heart failure related to coronary atherosclerosis, making it a leading cause of death and disability.
Mechanisms of Atherosclerosis-Related Ischemic Cardiomyopathy
Atherosclerosis causes myocardial dysfunction through several mechanisms. In its most advanced state, thrombotic closure of an epicardial conduit artery leads to downstream myocardial infarction and replacement of functioning cardiomyocytes with noncontracting fibrous tissue (◘ Fig. 8.3). Just short of infarction, gradual closure of the coronary arteries, usually accompanied by ingrowth of collateral vessels from neighboring coronary branches, leads to chronic cardiomyocyte ischemia. The chronic ischemia results in a noncontracting myocyte that eventually enters a hibernating state [8]. This results in impaired systolic function and impaired diastolic relaxation. Finally, atherosclerosis-associated abnormal vasomotion and constriction of the coronary arteries can lead to both transient loss of cardiomyocyte function and loss of myocytes in the subendocardium.


Fig. 8.3
Effects of coronary occlusion on the myocardium , as a function of the rate and severity of occlusion
Atherosclerosis is usually preceded by reduced endothelial control of vascular tone. Since arteries are prone to constrict in the absence of tonic NO production by the endothelium, these arteries are prone to inappropriate constriction at both the micro- and conduit vessel levels. For example, transient sympathetic stimulation that might cause an increase in contractility and blood flow can lead to microvascular constriction [see the heading below, “Stress-Induced Ischemic Cardiomyopathy”] in the face of increased oxygen demand due to the elevated contractile state. This can result in transient myocardial ischemia and dysfunction but usually does not lead to chronic heart failure or loss of pump function.
As atherosclerotic deposits develop within the coronary arteries, the usual initial response to increased coronary wall thickness is for the artery to grow externally (i.e., increase its outer diameter) while preserving the lumen caliber. This “positive remodeling” described by Glagov et al. continues until the wall thickness is increased by about 40 % [9]. Thereafter, the lumen of the artery slowly diminishes in caliber, usually in an eccentric manner. As the conduit coronary lumen narrows, blood flow to the downstream cardiac muscle is maintained by dilation of the microcirculation. Typically, this downstream microvascular dilation is engaged when the cross-sectional area of the large coronary lumen is about 70 % reduced, compared to the original lumen caliber [10].
With this downstream autoregulation, blood flow at rest is preserved. When myocardial oxygen requirements increase, however, capacity for further dilation is limited. This results in demand-induced ischemia. Typical demands that result in ischemia are exercise and elevated heart rate. Although elevated blood pressure also invokes more oxygen demand, it is accompanied by increased driving pressure for coronary flow through the stenotic lesion, which mitigates the effects of the stenosis.
As the arterial lumen narrows further, blood flow at rest diminishes because the downstream microcirculation is fully dilated and cannot further compensate for the upstream obstruction to flow. This occurs when 90 % of the arterial lumen cross-sectional area is obstructed. On the arteriogram, these lesions appear to cause subtotal occlusion of the epicardial artery, and the distal flow of contrast media is slow.
The effects of an upstream stenosis on myocardial blood flow can be mitigated by collateral blood vessel connections between the coronary branches. Small collateral arteries that usually connect the microcirculatory branches exist in most human hearts to a variable degree. The branches are most prominent in the subendocardium but can be found throughout the circulation [11]. In non-ischemic coronary beds, the available collateral blood flow is low: around 0.2–0.4 mL/g/min [12]. Repetitive ischemia can lead to the growth in diameter of collateral blood vessels such that, in some patients, collateral blood supply can nearly reach the level of the original flow from the epicardial artery [13]. Diabetics and patients with microcirculatory disorders appear to have less collateral blood flow [14].
Collateral blood flow can increase rapidly. Typically, hibernating myocardial segments, where the initial coronary occlusion did not result in infarction, have markedly better collateral blood flow. After sudden coronary occlusion, 48 % of patients have angiographically visible collateral support of the occluded coronary risk area at 6 h, whereas by 1–30 days, 92 % had angiographically visible collateral blood flow to the infarct risk area [15].
Physiology of Acute Myocardial Infarction and Relation of Ventricular Function
Most myocardial infarctions are due to a relatively sudden occlusion of an epicardial coronary artery at a site of atherosclerotic plaque rupture. The muscle zone fed by that artery is referred to as the “area at risk.” The larger the risk area, the larger the infarction, and the worse the outcome. Increases in the infarct volume bring a stepwise increase in long-term mortality. Infarction of more than 40 % of the left ventricular mass is generally not survivable [16].
Assessing the impact of therapies on myocardial infarction center on risk area measurements: Effective therapies change the ratio between the area at risk and the area of infarction. Cardiac magnetic resonance imaging (CMRI) with T2 weighting enables measurement of the edema that occurs in the area at risk after a prolonged ischemic episode. Delayed gadolinium (Gd) imaging , where the Gd contrast agent clears slowly from injured myocytes (early infarction) and fibrotic areas (late infarction), allows measurement of the infarct area. Using the two methods, an infarct-to-risk area ratio can be obtained.
Mechanisms of Infarction and Preconditioning
When atherosclerotic plaques rupture, the luminal platelets are activated by the collagen and other clot promoters in the plaque, such as tissue factor. This leads to intraluminal thrombosis, which can be highly variable. Patients with enhanced platelet function (such as those with inflammatory diseases or cigarette smokers) develop greater clot volume than others. Pieces of the intraluminal thrombus often break off and embolize downstream, leading to microinfarctions throughout the thickness of the downstream muscle. Activated platelets generate potent vasoconstrictors, such as thromboxane A2 and serotonin, leading to transmural ischemia from microvascular constriction downstream and spasm in the epicardial artery. Moreover, the thrombus in the artery is dynamic. Plasmin gradually dissolves the fibrinogen holding the platelets together, leading to thrombus resorption. Additional platelet activation leads to more clot deposition. As a result, arteries often open and close actively.
All of these factors lead to recurrent bouts of myocardial ischemia.
The ischemic episodes can result in ischemic preconditioning. In clinical studies, ischemic preconditioning can occur quite rapidly. For example, a 1-min coronary occlusion with an angioplasty balloon causes brief preconditioning to subsequent coronary occlusion. The time to ischemic changes showing on an electrocardiogram (ECG) lengthens with each subsequent balloon occlusion. Similarly, patients with prior intermittent angina before infarction have a better outcome and smaller infarction for the area at risk, presumably aided by preconditioning.
When an epicardial coronary artery becomes occluded suddenly, the ischemia is most profound in the subendocardial layers. Infarction occurs first in these layers and proceeds out to the mid- and subepicardial layers with prolonged occlusion. The periphery of the risk area is usually better supplied with nearby collateral blood flow from the surrounding unobstructed arteries. If, however, the surrounding arteries are also occluded or severely narrowed, the availability of collateral blood flow will be diminished, leading to a more extensive and dense infarction in the area at risk.
Left Ventricular Remodeling
Advanced IschCM leads to remodeling of the left ventricle. The muscle in infarcted areas has reduced or no contraction, leaving the remainder of the myocardium to sustain stroke volume. As a result of reflexive changes and increased filling pressures, the surrounding non-infarcted muscle becomes hypercontractile. Reduced pump capacity activates the sympathetic nervous system (both neural and through release of catecholamines from the adrenal glands). The prolonged increased load on the remaining muscle can lead to hypertrophy. In many cases, however, the blood supply of the surrounding muscle is also impaired. The increased load on the non-infarcted muscle can lead to chronic ischemia and worsening of the heart failure through deterioration of the contraction of the surviving viable muscle.
Over time, the left ventricle dilates, changing from a prolate ellipsoid to a spherical chamber. This increases the wall stress needed to develop a given intraventricular pressure, further increasing the workload of the remaining myocytes. Unlike many non-ischemic cardiomyopathies, IschCM is usually associated with segmental, nonhomogeneous loss of LV contraction. This further reduces ventricular efficiency by creating “hinge points” between contracting and noncontracting segments [17].
Regional loss of contraction can particularly affect mitral valve function. Akinesis in the areas supporting the papillary muscles leads to mitral regurgitation due to impaired systolic mitral closure. Like all dilated cardiomyopathies, dilation of the ventricle leads to an enlargement of the mitral annulus and reduced tethering of the mitral leaflets (the ventricle enlarges more than the mitral chordae lengthen), leading to mitral insufficiency. The degree of mitral regurgitation (MR) is affected substantially by changes in LV volume and geometry—and it can be quite dynamic.
Eventually, the downward spiral of left ventricular dilation, sympathetic overdrive, secondary left atrial dilation (often inciting atrial fibrillation), pulmonary congestion leading the pulmonary hypertension and right ventricular failure, and fluid retention from inadequate renal perfusion results in death .
Imaging in Ischemic Cardiomyopathy
Imaging can give important information about ventricular and valvular function , myocardial blood flow, changes in tissue histology, and, more recently, cardiomyocyte biochemistry. Much of this is discussed in Chaps. 5, 12, and 14. In general, the prognosis of ischemic cardiomyopathy is a function of the volume of infarcted muscle, expressed as a percentage of the myocardium; the volume of hibernating muscle; and the volume of muscle that becomes ischemic under stress conditions. Each of these parameters can be imaged using a variety of methods.
Assessment of Ischemia, Hibernation, and Infarction
Detection of Inducible Ischemia
The prognosis of all patients with coronary artery disease is related to the presence and volume of myocardial ischemia that can be induced under a stress condition. Whether ischemia is detected by ischemic electrocardiographic changes during stress (ST segment depression), loss of contraction on echocardiographic stress imaging, or a focal reduction in ventricular perfusion (nuclear imaging), the greater the volume of ischemic muscle and the less stress required for induction of ischemia, the worse the prognosis [18]. Not surprisingly, most studies show that the presence and extent of inducible ischemia also predicts, in part, the prognosis of patients with IschCM [19, 20].
Assessment of Fibrosis and Viability
A simple but insensitive and somewhat nonspecific method for detecting myocardial scar is the presence of Q waves on the electrocardiogram [21]. Initial determination of the volume and location of infarction-related fibrosis is derived from X-ray contrast ventriculography or standard echocardiography. Akinetic areas are assumed to be fibrotic, particularly if wall thinning or loss of trabeculation could be detected.
Identifying myocardial hibernation, however, makes clear that simple assessments of contractile state do not accurately reflect the underlying viability or presence of cardiomyocytes (as opposed to scar). Moreover, the area and transmural depth of fibrosis after myocardial infarction varies widely after myocardial infarction. The presence of collateral blood flow to the area at risk, the occurrence and timing of reperfusion of the infarct-related artery, and ischemic preconditioning prior to coronary occlusion all impact the extent of the infarction and subsequent fibrosis. Hence, some areas might be densely infarcted, whereas others have patchy or only a subendocardial-layer scar.
The extent of myocardial focal scar can be assessed indirectly by nuclear (201-Tl and 99-Tc agents) imaging, where the absence of tracer uptake (on immediate and late imaging) implies the presence of scar. The spatial resolution of these methods, however, limits their utility for precise quantitative assessment [22]. Positron emission tomography (PET) imaging [see below] improves spatial resolution but still has limitations for precise transmural volumetric assessment .
The most accurate method for assessing the volume and distribution of myocardial fibrosis is MR imaging after gadolinium-based contrast agent infusion. Gd agents wash out slowly from fibrotic tissue and can be imaged after the initial perfusion scan is obtained. Survival is tightly linked to the fraction of the ventricle that contains scar tissue; the greater the volume of scar, the greater the mortality risk (◘ Fig. 8.4).


Fig. 8.4
Survival of patients with ischemic cardiomyopathy as a function of the amount of scar tissue (expressed as a percentage of the left ventricular mass) measured using late Gd enhancement on MR imaging. Source: Kwon et al. Circulation. 2012;126(11 Suppl 1):S3–S8 [55]
Myocardial viability can be assessed indirectly by the muscle response to stimulus intended to evoke enhanced contraction, or it can be inferred from noncontractile myocardium that is not fibrotic.
Horn et al. identified a subset of patients with reduced ventricular function who had improved contraction during epinephrine infusion (a so-called epinephrine ventriculogram ) [23]. In addition, it was observed that the contraction after a premature ventricular contraction (PVC)—with its compensatory pause and heighted inotropic state—is enhanced. This enhanced contraction with potentiation could be imaged easily during left ventricular angiography. Increased contraction in an akinetic or hypokinetic zone was predictive of improved function after revascularization [24, 25]. This led Diamond et al. to coin the term hibernation to describe the phenomenon [26].
Noninvasive approaches to assessing viability in noncontractile zones have centered on perfusion imaging. Initially, 201thallium agents or 99technetium agents are initially taken up by cardiomyocytes in proportion to blood flow. Ischemic muscle appears as a perfusion defect on single photon emission imaging. Over time, however, these agents are concentrated by the cardiomyocytes—and concentrated by myocytes even in the presence of ischemia. Repeat imaging after a 4- to 24-h interval allows the ischemic muscle to concentrate 210Tl. Scar does not concentrate 201Tl. If a perfusion defect on the initial scan fills in on late imaging, the area can be assessed as viable but ischemic. Occasionally, a second dose of radiotracer is given to intensify late enhancement of the ischemic zone.
Nuclear imaging was improved by adding PET agents that enabled more accurate assessment of myocardial perfusion, better spatial resolution, and, also, direct assessment of myocardial metabolism. Imaging with 82Rb, 14N ammonia, or 15O water enabled progressively better measurements of myocardial blood flow with better spatial resolution than the older 201Tl- or 99Tc-based compounds. Assessment of glucose metabolism using fluorodeoxyglucose (18FDG) permitted imaging of cardiac muscle that has switched from fatty acid (oxidative) metabolism to glucose as an energy source (anaerobic metabolism). Areas with reduced blood flow and preserved anaerobic metabolism indicate ischemic but viable myocardium.
Subsequently, the response to catecholamine stimulation, usually by intravenous dobutamine, during echocardiographic imaging was developed as a test of contractile reserve [27]. The normal response to dobutamine infusion is a stepwise improvement in contraction and ventricular ejection fraction. Ischemic but viable noncontracting muscle typically has a two-phase response. Initially, the contraction improves. As the stimulant dose is increased, however, the segment again become dysfunctional. Nonviable myocardium either has no significant contractile response or becomes dyskinetic.
Although these indirect methods of assessing viability have some accuracy in detecting muscle that will improve function with revascularization, they are only modestly accurate [28, 29] (◘ Fig. 8.5). MR imaging allows much better special resolution of perfusion by the flow of imaging Gd-based contrast agents within the myocardium. Gd agents are taken up slowly and released slowly from the extracellular space of fibrous tissue, allowing excellent spatial identification of scar. Areas with reduced perfusion and the absence of scar are, in theory, viable. In one study, severely hypokinetic or akinetic myocardium without scar (i.e., no hyper-enhancement with Gd agents) had 86 % and 100 % incidence, respectively, of improved contraction after revascularization [30].


Fig. 8.5
Receiver operating curve showing the diagnostic accuracy of different techniques to assess myocardial viability. DSE = dobutamine stress echocardiography; DSMR = dobutamine stress magnetic resonance; FDG = fluorodeoxyglucose; LGE = late gadolinium enhancement; MIBI = methoxyisobutylisonitrile. Source: Schuster et al. J Am Coll Cardiol. 2012;59(4):359–70 [29]
Viable but akinetic myocardium is quite common in patients with ischemic cardiomyopathy, but the volume of hibernating muscle varies considerably. One major advantage of MR imaging is that the transmural distribution of blood flow and fibrotic tissue can be assessed quantitatively. Most infarctions are not transmural. The thickness of the infarction has a great impact on the ability of the myocardial segment to return to meaningful function after revascularization. Segments with less than 25 % wall thickness scar usually improve in function significantly. Those with >50 % scar usually remain akinetic [30] (◘ Fig. 8.6).


Fig. 8.6
Relation between the transmural extent of hyper-enhancement before revascularization and the likelihood of increased contractility after revascularization. Source: Kim et al. N Engl J Med. 2000;343(20):1445–53 [30]
Using MR to assess viability can be enhanced by administering dobutamine to determine if wall motion improves in an akinetic section—in a manner similar to dobutamine stress echocardiography. The advantage of MR imaging is that it is three-dimensional and offers greater spatial resolution. In addition, the use of strain imaging may improve the assessment of viability [31].
Complications of Ischemic Cardiomyopathy
Infarction-Related Aneurysm
A full thickness scar can develop in densely infarcted areas, leading to aneurysm formation and bulging of the LV chamber during systole (◘ Fig. 8.7). This reduces LV efficiency and provides an area of blood stasis that often leads to thrombus formation [36]. The efficacy of anticoagulation therapy is unclear. In one recent report, warfarin anticoagulation did not appear to be effective [37]. In addition, the rim of the aneurysm can be the substrate for recurrent ventricular tachycardia and sudden death. Most aneurysms are in the perfusion field of the mid or distal left anterior descending artery, although they can occur anywhere in the ventricle. Aneurysms located near the papillary muscles’ mitral apparatus can cause mitral regurgitation.


Fig. 8.7
An apical left ventricular aneurysm (arrows) due to a prior myocardial infarction in the left anterior descending territory is shown from a postmortem examination
Patients with a large, discrete, dyskinetic aneurysm and heart failure symptoms or thrombus in the aneurysm may benefit from surgical aneurysmectomy to improve ventricular efficiency and reduce the likelihood of thromboembolism. In addition, ventricular arrhythmia that cannot be controlled with ablative therapies can be treated with aneurysm resection.
Percutaneous placement of the Parachute device, which occupies the aneurysmal cavity, may also be effective in reducing symptoms from a discrete apical aneurysm [38]. Thrombus within the aneurysm is a contraindication .
Embolism
Although all cardiomyopathies have a higher risk of cardioembolic events (primarily stroke), ischemic cardiomyopathy has a particularly elevated risk for several reasons. First, the presence of dyskinetic myocardium can lead to blood flow stasis and clot formation. Second, ischemic heart disease is often a disease of coronary thrombosis. Many patients with ischemic cardiomyopathy have a greater propensity to clot.
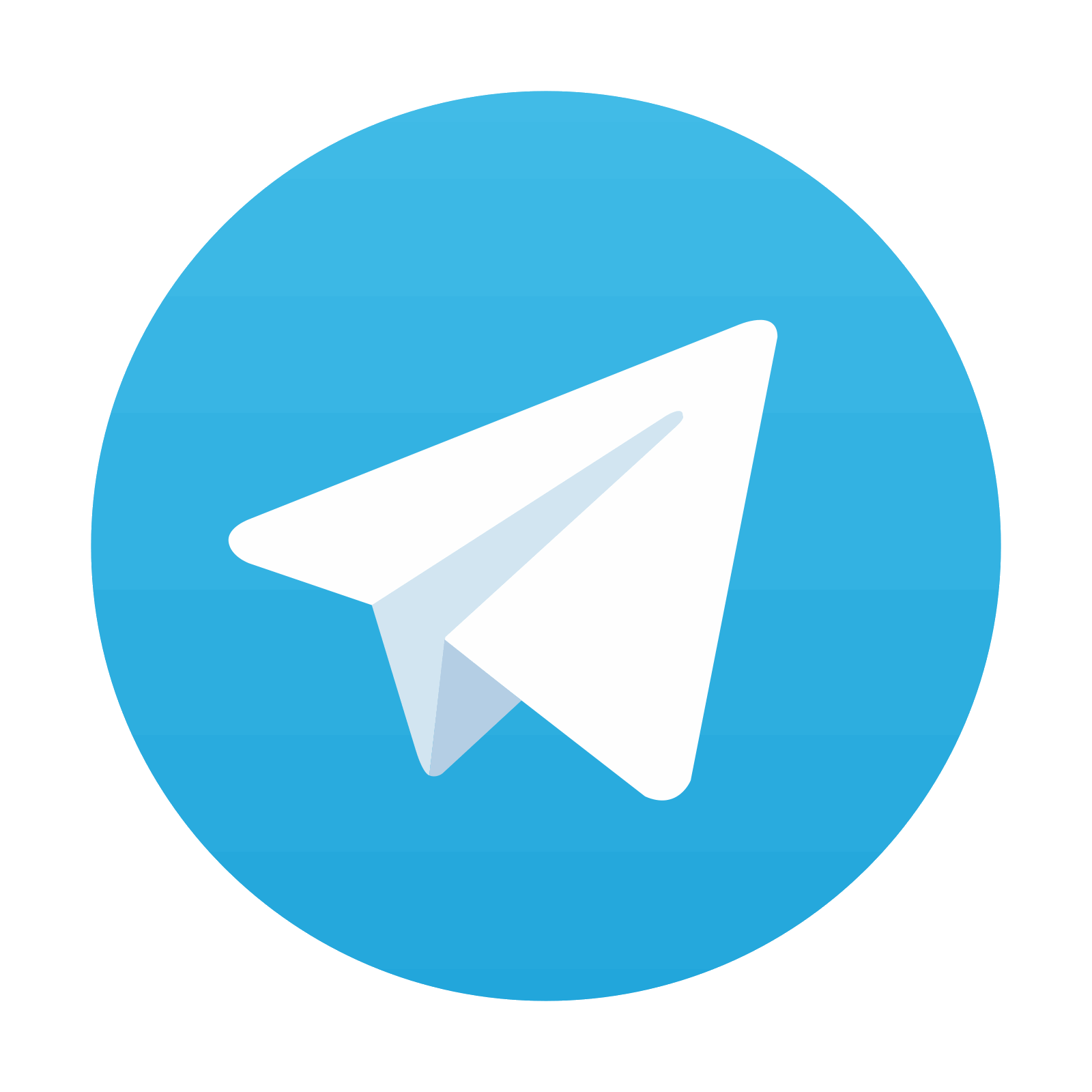
Stay updated, free articles. Join our Telegram channel
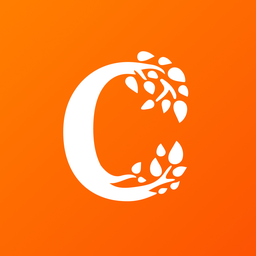
Full access? Get Clinical Tree
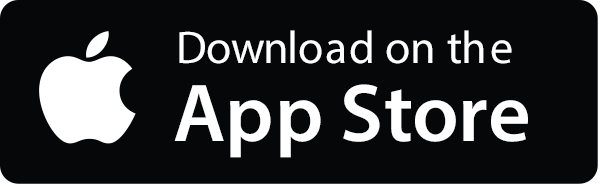
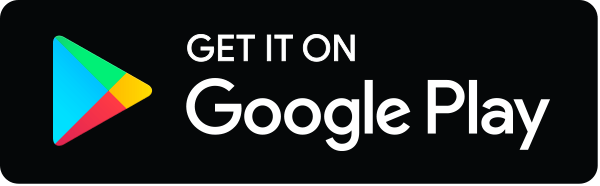