© Springer Science+Business Media New York 2015
James R. Klinger and Robert P. Frantz (eds.)Diagnosis and Management of Pulmonary HypertensionRespiratory Medicine1210.1007/978-1-4939-2636-7_1717. Investigative Therapies in Pulmonary Arterial Hypertension
(1)
Pulmonary Diseases and Critical Care Medicine, Division of Pulmonary and Critical Care, Pulmonary Hypertension Center, University of South Alabama, Mastin Professional Building, 2451 Fillingim Street, Mobile, AL 36617, USA
Abstract
Pulmonary arterial hypertension (PAH) remains a serious, life threatening disease of unclear etiology. Despite the rapid development of numerous drugs to treat the disease, no cure is presently available. New treatments for PAH that are able to reverse the abnormal pulmonary vascular remodeling that is responsible for much of this disease are badly needed. To accomplish this goal, novel therapies that target many of the dysfunctional pathways that have been identified in PAH will need to be developed. This chapter reviews many of the known alterations in gene expressions, vasoconstriction, inflammation, metabolism, and cellular proliferation that have been identified in the pathogenesis of PAH. Potential pharmacologic targets arising from these abnormalities are reviewed along with data, where available, from animal studies and small clinical trials that have attempted to treat pulmonary vascular disease through manipulation of these pathways.
Keywords
Pulmonary arterial hypertensionInvestigational therapyGene therapyCell therapyBone morphogenic protein receptor 2Abbreviations
5HT
Serotonin
ACE
Angiotensinogen-converting enzyme
ALK-1
Activin like kinase 1
AT III
Angiotensin III
AT-1
Angiotensinogen receptor-1
AT-2
Angiotensinogen receptor-2
BMPR2
Bone morphogenetic protein receptor 2
CML
Chronic myelogenous leukemia
COPD
Chronic obstructive lung disease
DCA
Dichloroacetate
DHEA
Dehydroepiandrosterone
EIF2AK4
Eukaryotic translation initiation factor 2 alpha kinase 4
eNOS
Endothelial nitric oxide synthase
EPCs
Endothelial progenitor cells
HDAC
Histone deacetylase
HIF1α
Hypoxia inducible factor 1α
IL-1
Interleukin-1
IL-13
Interleukin-13
IL-6
Interleukin-6
KCNK3
Potassium channel subfamily K member 3
Kv
Voltage-gated potassium channels
miRNA
MicroRNA
MSC
Mesenchymal stems cells
NFAT-1
Nuclear factor of activated T-cells 1
NO
Nitric oxide
PAH
Pulmonary arterial hypertension
PASMC
Pulmonary artery smooth muscle cells
PDGF
Platelet-derived growth factor
PGI2
Prostacyclin
PH
Pulmonary hypertension
PPAR γ/β
Peroxisome proliferator-activated receptors γ/β
RAAS
Renin–angiotensin–aldosterone system
RV
Right ventricle
SERCA 2a
Sarcoendoplasmic reticulum calcium transport ATPase 2a
siRNA
Small interfering RNA
SOD2
Superoxide dismutase 2
TGF β
Transforming growth factor β
TKI
Tyrosine kinase inhibitor
TRPC
Transient receptor potential channels
TXA2
Thromboxane
VEGF
Vascular endothelial growth factor receptor
VEGFr
Vascular endothelial growth factor receptor
VIP
Vasoactive intestinal peptide
Introduction
Despite the availability of multiple therapies specifically approved by regulatory agencies for the treatment of pulmonary arterial hypertension (PAH), the diagnosis remains indicative of a progressive, life-limiting process resulting in substantial morbidity and mortality. Identification of treatments aimed to normalize life expectancy in these patients is a significant priority and to date no single therapy for PAH can achieve this goal. This is not at all surprising given the multifactorial nature of the pathogenesis of PAH and the clinical heterogeneity of patients classified as World Health Organization Group 1 PAH. Future effective treatments for PAH may ultimately rely on identification and development of multi-targeted strategies that go well beyond our current therapeutic targets. It is impossible to summarize all the potential therapies that are being evaluated for PAH. Focusing on important pathologic processes in PAH and investigative therapies related therein may provide an important approach to identify integrative, complementary, pluripotent therapies for future consideration [1].
Potential Therapeutic Targets for PAH
The pathophysiology of PAH is complex with many potential targets that have been identified through various studies including genomic approaches, clinical investigations, and preclinical animal studies. There are equally numerous ways to categorize these targets. For purposes of this review, selected targets for future treatments will be considered. Additionally, most current and future treatments are aimed at reversing the abnormal structure and function of the pulmonary circulation. Enhancing right ventricle (RV) function is also an important consideration in improving patient functional status and outcome.
Genetic and Epigenetic Targets
Identification of bone morphogenetic protein receptor 2 (BMPR2) mutations as a cause of familial PAH in 2000 heralded over 14 years of research devoted to understanding how the wide variety of mutations cause PAH. Haploinsufficiency, dominant negative effects, and abnormal signaling through downstream pathways have all been associated with PAH. Because of these variable effects of the BMPR2 mutation, the approach to restoring normal gene expression and function of BMPR2 is daunting. What is clear is that developing ways to increase expression of the normal BMPR2 allele may result in substantial benefit. Gene therapy via adenoviral gene transfer in animal studies has demonstrated benefit of increasing expression of the normal BMPR2 [2]. However, gene therapy in humans with PAH is significantly in the future. Alternative strategies, aimed at increasing BMPR2 expression and activity, have led to several new lines of clinical investigation. Recently, FK506 (tacrolimus) has been identified as a potential potent inducer of BMPR2 activity. Its effect on BMPR2 is only partially explained by inhibition of calcineurin and nuclear factor of activated T-cells 1 (NFAT-1) signaling. Tacrolimus was also able to improve endothelial cell function in cells derived from PAH patients as well as decrease pulmonary hypertension (PH) in hypoxic mice with BMPR2 haploinsufficiency and rats with monocrotaline and vascular endothelial growth factor receptor (VEGFr)/hypoxia-induced PAH [3].
Other targets aimed to increase BMPR2 expression and activity by acting as molecular chaperones and increasing transcription have been proposed. Ataluren increases ribosomal transcription of genes with stop codon mutations [4] and has been tested in several genetic diseases with mixed results. Ongoing trials in patients with cystic fibrosis are taking place now [5] and may point to a possible option in PAH patients with specific BMPR2 mutations in the future. Some mutations of BMPR2 are associated with protein folding abnormalities that do not allow BMPR2 trafficking out of the endoplasmic reticulum and several different chaperones to move the mis-folded protein to the cell surface have been studied [6].
While mutations in BMPR2 are most commonly associated with PAH, other genes such as activin-like kinase 1 (ALK-1), endoglin, potassium channel subfamily K member 3 (KCNK3), eukaryotic translation initiation factor 2 alpha kinase 4 (EIF2AK4), and voltage-gated potassium channels (Kv’s) that are mutated or abnormally expressed in PAH may also benefit from the approaches above. Additionally, abnormal activity of BMPR2 signaling may also alter the expression of endogenous regulators of the lung circulation. One such example is apelin, a peptide that is implicated in endothelial and smooth muscle proliferation and vasodilation, which is reduced by mutations in BMPR2 that disrupt peroxisome proliferator-activated receptors γ/β (PPAR γ/β) signaling, thus providing some rationale for the therapeutic potential of PPAR agonists in PAH [7–9]. PPAR agonists are also associated with attenuation of PH in animal models by modulating the levels of other vasoactive mediators such as endothelin-1 and VEGF [10].
Other epigenetic changes are also found in PAH patients. One area of increasing interest is the role of micro RNAs (miRNAs) and small interfering RNAs (siRNAs) to regulate gene expression in PAH. Transfection of RNA containing vectors to enhance or inhibit gene expression has been proposed as potential future therapies as increasing evidence supports the role of miRNA and siRNA in PAH. A recent review highlighted many of the RNAs implicated in PAH with additional RNA targets reported every year [11].
DNA modifications such as increased methylation lead to changes in expression of the gene. An example is the methylation of superoxide dismutase 2 (SOD2) by methyltransferases resulting in decreased SOD expression, changes in redox state and increased expression of pro-proliferative signals such as hypoxia inducible factor 1α (HIF1α) [12, 13]. Modification of histones by acetylation in PAH has also been proposed as an important epigenetic phenomenon. Inhibition of de-acetylation by inhibitors of histone deacetylase (HDAC) causes changes in gene expression that result in inactivation of some genes and activation of others. In PAH, studies in pulmonary artery smooth muscle cells (PASMCs) and several animal models of PH have demonstrated a decrease in PASMC proliferation and inflammation associated with targeted inhibitors of HDAC [14]. Inhibitors of HDAC have also been used in studies looking at RV function in models of PAH with mixed results. In left ventricular hypertrophy, inhibitors of HDAC were beneficial, whereas in studies of different models of PAH and with different HDAC inhibitors, effects on the RV were beneficial (in compensated RV hypertrophy and monocrotaline-induced PH) but were associated with RV failure in others [15, 16].
Manipulating the expression of genes implicated in PAH is a promising area of active investigation. Hopefully, restoration of more normal gene expression and ultimately more normal cell signaling will restore normal pulmonary vascular and RV function and improve the life expectancy in PAH patients.
Vasoconstriction and Vasoactive Targets
Enhanced pulmonary vasoconstrictive responses to a wide variety of stimuli have been identified in PAH. Indeed, all currently available PAH treatments directly target vasoconstrictive pathways.
Vasoconstriction of vascular smooth muscle requires an influx of calcium in order to activate the contractile machinery. Calcium can be released from intracellular stores or imported from the extracellular environment. Transient receptor potential channels (TRPC) regulate the release of calcium from intracellular stores. Increased activity of TRPC 6 has been identified in PAH patients and is associated with a single-nucleotide polymorphism [17]. Increased expression of TRPC 6 was also associated with enhanced proliferation of PASMCs [18]. Loss of TRPC 4 channel activity in rats is associated with improved survival in models of PAH [19]. The sarcoendoplasmic reticulum calcium transport ATPase 2a (SERCA 2a), a channel that regulates release of calcium from the sarcoplasmic reticulum, has also been implicated in PAH [20]. Modulating the influx of calcium has been a target of PAH therapies by directly inhibiting the entry of calcium through T- or L-type channels using calcium channel blockers. However, the clinical effectiveness of these alone has been modest at best and more selective regulators of calcium entry such as the channels above may provide important treatment targets [21].
Calcium influx occurs when the smooth muscle cell is depolarized. In PAH, several voltage-gated potassium channels (Kv) have been implicated. Down regulation of several potassium channels, most notable Kv1.2 and 1.5 are associated with membrane depolarization and calcium influx. Maneuvers that increase Kv expression and function (by gene transfer, dichloroacetate, etc.) reduce experimental PH by causing cellular hyperpolarization [22]. Recently, the KCNK3 gene that encodes potassium channel subfamily number 3 has been identified as a cause of heritable PAH in several families. Mutations in this channel lead to a channelopathy characterized by loss of potassium channel function and hyperpolarization of smooth muscle cells [23]. This finding definitively links abnormal potassium channel function to PAH and restoring normal channel function is a viable strategy for future consideration.
Vasoconstriction can also be achieved by a variety of vasoactive molecules that interact with cell surface receptors and cause vasoconstriction through a variety of intracellular signaling processes. Current treatments including endothelin-1 receptor blockade or increasing levels of prostacyclin-generated cAMP and nitric oxide-generated cGMP \take advantage of this strategy to lessen or enhance downstream signals respectively. There are many other molecules that modulate vasoconstriction including vasoactive intestinal peptide (VIP), thromboxane (TXA2), angiotensin III (AT III), apelin, serotonin (5HT), etc. all of which may cause pulmonary vasoconstriction through several different pathways. VIP inhibits vasoconstriction and also acts to vasodilate the circulation. Decreased VIP expression results in worse PH in animal models, and treatment with exogenous VIP attenuates this effect [24]. However, despite positive results from early clinical trials, VIP administration in PAH patients did not result in measureable improvements [25]. 5HT has long been associated with PAH and interference with the serotonin transporter has been demonstrated to decrease PH in animal models [26]. Terguride, is a 5HT transporter inhibitor, that was not found to be effective in the treatment of PAH in a preliminary phase IIa study [27], but continues to be investigated in combination with other drugs. Apelin, as described above is decreased in PAH due to mutations in BMPR2. It has many effects on the pulmonary circulation and dilates the lung circulation of animals with PH possibly through its effects on altering expression of endothelial nitric oxide synthase (eNOS) to increase nitric oxide (NO) production. Apelin administration has been associated with decreased PH in animal models [28].
Activation of Rho kinase in PAH leads to sustained contraction in PASMCs through “Ca sensitization” of the contractile apparatus, namely increased levels of phosphorylated myosin light chain [29, 30]. Inhibitors of Rho kinase, both direct and indirect, result in substantial reversal of vasoconstriction and vascular remodeling in several animal models of PH [31–36]. Several small scale human clinical trials of acute administration of the direct Rho kinase inhibitor fasudil in PAH patients demonstrated acute pulmonary vasodilatory effects [37–40]. Long-term clinical trials of direct Rho kinase inhibitors have not yet been carried out. Indirect inhibitors of Rho kinase, such as dehydroepiandrosterone (DHEA), are attractive candidate drugs that have been associated with attenuation and reversal of PH in animal models [41] and improved exercise capacity in patients with chronic obstructive lung disease (COPD) associated PH [42].
Identification, testing and production of specific inhibitors of the above mentioned agents (many of which are implicated in PAH), are daunting. A strategy that identifies important intracellular signaling hubs common to several important players in the pathogenesis of PAH may prove more effective than targeting pathways individually.
Inflammation and Inflammatory Mediator Targets
Inflammation that sets in motion a series of events that promote vascular dysfunction and remodeling has long been hypothesized to be an important contributor to the development of PAH [43]. The association of PAH with autoimmune and inflammatory diseases is a significant part of this hypothesis. However, immunosuppressive therapies have had limited effectiveness in the treatment of PAH. The pro-inflammatory cytokines, interleukin-1 (IL-1) and -6 (IL-6), are increased in PAH patients and inhibition of these is associated with decreased PH in animal models. IL-13 and signaling via transforming growth factor β (TGF β) is additionally associated with schistosomiasis-related PAH [44]. Several animal studies and case reports have found that inhibition of IL-6 is associated with decreased PH [45]. The anti-IL-6 monoclonal antibody has been associated with decreased pulmonary vascular disease in a patient with PAH associated with connective tissue disease and may represent a future therapeutic option for some PAH patients [46]. Cell-mediated immune functions have also been implicated in PAH and may represent important therapeutic targets. Patients with human HIV and athymic rats are more susceptible to PH suggesting an important role of T-cells and chronic inflammation in PAH [47, 48]. Given the relationship to autoimmune diseases, B-cell activation may play a role in PAH suggesting the potential option for anti-B-lymphocyte antigen CD20 treatment in the future. Several case reports suggest that rituximab may be helpful in patients with PAH associated with connective tissue disease [49, 50].
Vascular Cell Proliferation and Vessel Remodeling Targets
Excessive proliferation of cells in the walls of the pulmonary arteries and formation of the plexiform lesion is a hallmark of severe PAH. Events leading to the alteration of the normal vascular structure likely are multifactorial involving biochemical and mechanical forces that promote cell proliferation and angiogenesis. Many molecules known to be important in PAH such as NO, 5HT, TXB2, Rho kinase, and prostacyclin (PGI2). have direct effects on vascular tone, but also contribute to the proliferative phenotype. Thus, manipulation of these agents may help to decrease or reverse pulmonary vascular remodeling in addition to decreasing pulmonary vascular tone.
More direct approaches to address vascular remodeling target specific growth factor pathways and the extracellular matrix. Several important growth factors have been implicated in PAH. Platelet derived growth factor (PDGF) has been implicated in the proliferation of pulmonary vascular cells. Imatinib, a tyrosine kinase inhibitor (TKI) that inhibits the PDGF receptor, has been the most widely studied tyrosine kinase inhibitor in PAH. Imatinib was originally developed to inhibit the pro-oncogenic tyrosine kinase Bcr-abl in chronic myelogenous leukemia (CML). Given its ability to inhibit PDGF receptor and proto-oncogene c-Kit, imatinib was proposed as a potential regulator of PAH-related vascular proliferation [51, 52]. Despite initial excitement in animal models, case reports and small clinical trials [53, 54], imatinib failed to achieve regulatory approval after a large-scale clinical trial identified important concerns regarding the risk to benefit ratio of the treatment [55]. Other TKIs that target PDGF have also had some encouraging preclinical success, but may have limited clinical usefulness owing to other less beneficial effects. For example, dasitinib, a TKI with broader inhibitory properties than imatinib, also approved for treatment of CML, has been associated with the development of PAH [56].
Other potential targets are TKIs targeting epidermal growth factor receptor (erlotinib, gefitinib) and multi-kinase inhibitors (sorafenib, sunitinib), but as was seen with imatinib, significant limitations to their use lie in unacceptable adverse events and side effects and limited efficacy in animal models [57, 58]. Given these contradictory clinical effects and substantial adverse events, the future of TKIs in PAH is uncertain [59].
Modulation of extracellular matrix in PAH may also be a potential target for future treatments. Increased activity of elastases located in the pulmonary vascular wall allows for degradation of the extracellular matrix and, by inducing expression of other molecules such as tenacin and fibronectin, promotes cell proliferation and vascular remodeling [60]. Elafin, an inhibitor of serine protease, decreases and reverses severe PH in animal models [61, 62] and will likely undergo clinical testing in the near future.
Metabolic Targets
Derangements in the energy metabolism of pulmonary artery cells in PAH are being characterized. Examination of the RV and lungs of patients and animals with experimentally induced PH demonstrate a wide array of changes in expression of genes engaged in metabolic processes [63, 64]. Several mitochondrial abnormalities have been found in pulmonary vascular cells and the RV that lead to anaerobic metabolism (glycolysis) instead of aerobic metabolism through activation of pyruvate dehydrogenase kinase. This glycolytic state then leads to decreased intracellular reactive oxygen species generation, increased HIF1α expression and activity, and ultimately activation of vasoconstrictive and proliferative signaling by decreasing Kv channel expression and activity [65]. Dichloroacetate (DCA), an inhibitor of mitochondrial pyruvate dehydrogenase has demonstrated reversal or reduction of PH in animal models [66–69] and is undergoing clinical testing in PAH patients. Oxidation of fatty acids also contributes to this glycolytic shift, and several inhibitors of fatty acid oxidation, trimetazidine and ranolazine, have demonstrated potential efficacy in animal models of PAH by reversing the shift toward glycolysis [70, 71] Ranolazine is currently being evaluated in PAH patients at a single clinical center.
Neurohormonal Targets
Much of the morbidity and mortality of PAH patients lies in the adaptation and function of the RV to the increased demands of downstream resistance. Clearly, developing effective treatments to decrease the resistance due to vasoconstriction and remodeling is the ultimate RV “specific” treatment. Short of this, improving adaptation of the RV is an important therapeutic consideration. Many of the proposed PAH treatments above additionally involve adaptations that are seen in the RV as well as pulmonary vasculature. Recently, attention to the neurohormonal activation in PAH patients has received increasing attention. The sympathetic nervous system and renin–angiotensin–aldosterone system (RAAS) are activated in PAH [72] and represent potential targets for treatment.
Sympathetic tone is increased in patients with PAH and adrenergic receptor expression is also reported as increased in the RV of patients with PAH right ventricles [73, 74]. Activation of this system was considered adaptive to sustain cardiac output by increasing contractility, heart rate, and systemic blood pressure, and thus, manipulation of adrenergic receptors was considered unsafe in PAH patients. However, sympathetic activation has been associated with increased mortality in PAH patients [75] which has led to a number of studies of selective β-receptor blockade in PAH. In several preclinical models of PAH, the α1/β1/β2-adrenergic receptor antagonist carvedilol and β1-selective receptor antagonist bisoprolol are associated with improved RV function [76–78]. A retrospective review of PAH patients revealed no difference in clinical outcomes in patients who received β-blockers compared to those who did not [79, 80] suggesting that the safety of β-blockade in PAH patients should be reconsidered [72].
Activation of RAAS is also found in patients with PAH [72, 81]. Several case series of patients with PAH hinted at efficacy of angiotensinogen converting enzyme (ACE) inhibition [82], but concerns regarding the potential for hypotension limited further investigations. More recently, approaches aimed at selective targeting of the angiotensin (AT) pathway have identified the potential therapeutic value of targeting AT receptor-1 (AT1) (using the AT-1 antagonist losartan) while keeping ACE and AT-2 function (both with purported vasodilatory effects) and signaling intact [81]. Additional support of this approach is demonstrated by the use of ACE-2 agonists to decrease pulmonary artery pressure and RV hypertrophy and dysfunction in animal models of PAH [83–85].
Aldosterone antagonism is a mainstay of left ventricular failure treatment [86], but its role in RV failure is less well understood. However, increased levels of aldosterone are found in PAH patients [87] and treatment with aldosterone antagonists is included in several recent treatment guidelines. Evidence for this recommendation is increasingly apparent as demonstrated in recent studies that identified that upregulation of aldosterone is associated with increased ET-1 and decreased NO availability which is reversed by aldosterone antagonists in animal models of PAH [88, 89]. Review of the ARIES data of the selective ET-1, a receptor antagonist ambrisentan in PAH, suggests a complimentary benefit when combined with the aldosterone antagonist spironolactone [90].
Lung Cell-Targeted and Cell-Based Therapeutic Targets
Therapies designed to repair and remodel the pulmonary arteries in PAH may be best approached using strategies to deliver pharmacologic agents and genes directly to the abnormal lung circulation or to repopulate the pulmonary vasculature with cells that have normal phenotypes and behaviors. Targeting molecules to the lung circulation can be achieved by a number of mechanisms including the use of chaperones, exploiting lung-specific cell surface receptors, targeting lung-specific gene expression and identification of lung-specific signaling pathways. Numerous examples of this strategy are being investigated including use of specific lung endothelial cell surface receptors, peptides that chaperone molecules to abnormal pulmonary endothelium, and RNAs that target lung-specific gene expression. By using lung-targeted approaches, the limitations of systemic delivery of therapeutics and potential systemic side effects may be limited [91].
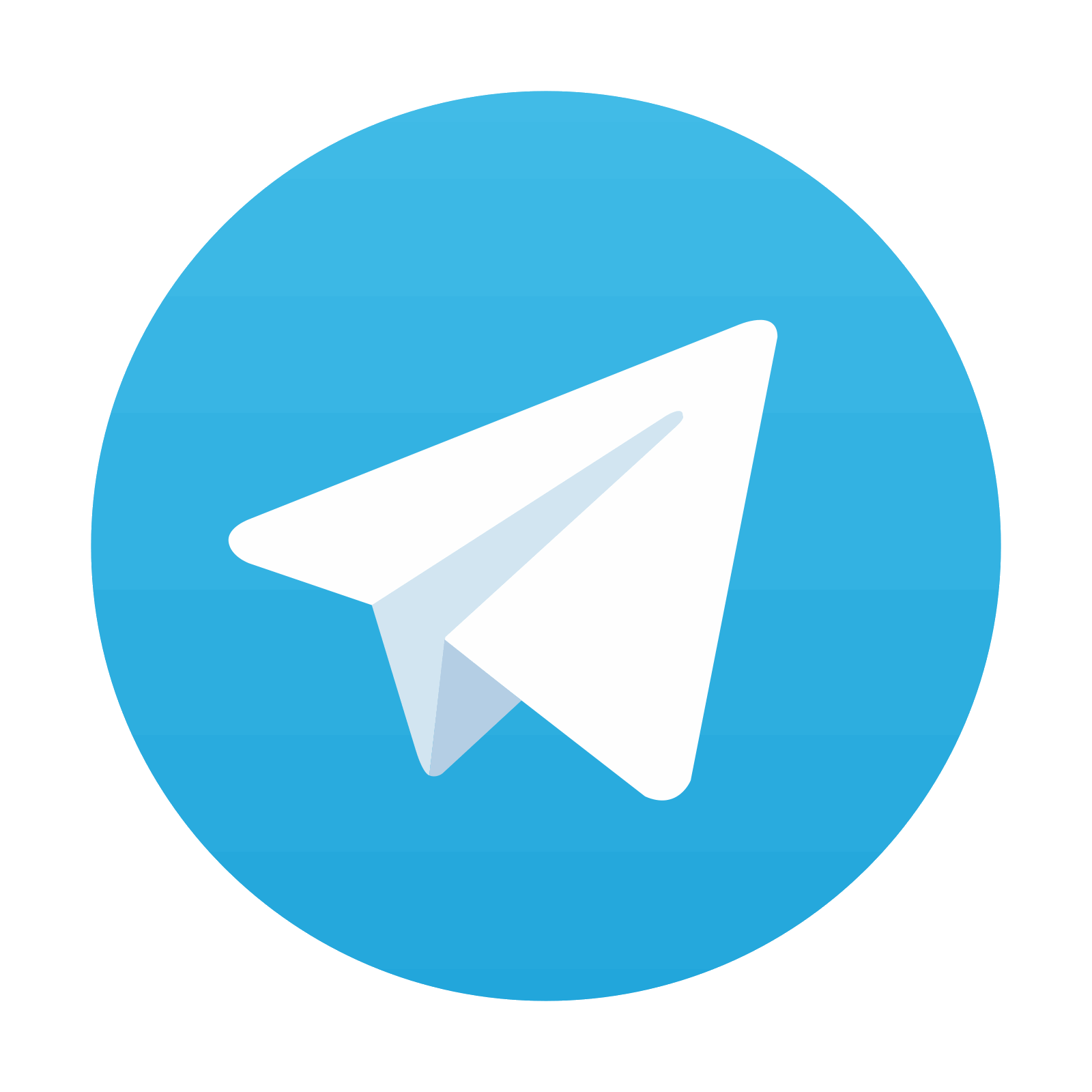
Stay updated, free articles. Join our Telegram channel
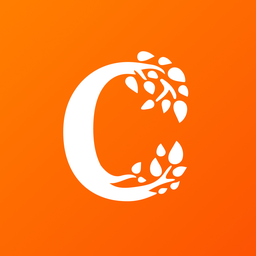
Full access? Get Clinical Tree
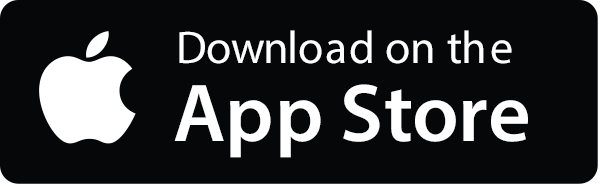
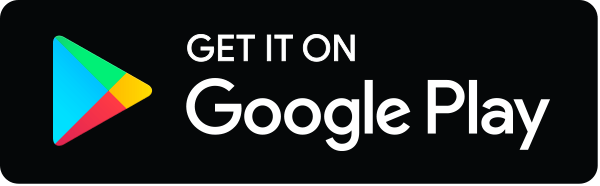