Chapter 29 Invasive Respiratory Support
Invasive ventilation is positive pressure ventilation applied via an endotracheal or tracheotomy tube. Most patients undergoing cardiac surgery do not have pulmonary disease and require invasive ventilation only until they have recovered from the surgery enough to be awakened. The ventilatory management of these patients is discussed in Chapter 17. In this chapter, the focus is on the care of patients with severe respiratory or cardiac disease who require prolonged and often complex forms of ventilation.
In the first part of the chapter the pathophysiologic effects of positive pressure ventilation are discussed. In the second part the principles of ventilator function are described. In the third part, ventilatory strategies in specific clinical situations, weaning ventilation, and tracheotomies are discussed. Because cardiothoracic intensive care units (ICUs) are often centers for mechanical cardiorespiratory support (see Chapter 22), a discussion of advanced ventilatory therapies, such as prone positioning and highfrequency oscillation, is also included. The indications for invasive ventilation are outlined in Chapter 27.
PATHOPHYSIOLOGY OF POSITIVE PRESSURE VENTILATION
In normal breathing, as the chest cavity expands during inspiration a negative pressure is generated within the alveoli, which draws air into the lungs. With invasive (or noninvasive) ventilation, positive pressure is applied to the airway and gas is blown into the lungs. Invasive ventilation is therefore nonphysiologic and has important respiratory and cardiac effects.1
Respiratory Effects
Gas Exchange
In patients with normal lungs, anesthesia and positive pressure ventilation increase ventilation-perfusion (V/Q) mismatch, which slightly impairs gas exchange. This is caused mainly by changes in the distribution of ventilation. In normal breathing, both ventilation and perfusion are greatest in the dependent regions of the lung, but in positive pressure breathing, ventilation is distributed more evenly throughout the lung. Thus, there is relatively more perfusion to dependent regions, which increases intrapulmonary shunt (a low V/Q ratio), and more ventilation to nondependent regions, which increases alveolar dead space (a high V/Q ratio). Intrapulmonary shunting reduces arterial oxygen tension (Pao2) whereas alveolar dead space increases arterial carbon dioxide tension (Paco2; see Chapter 1). Intrapulmonary shunting also occurs if functional residual capacity (FRC) is less than closing capacity, as occurs during anesthesia (see Chapter 1). Intrapulmonary shunting is partly ameliorated by positive end-expiratory pressure (PEEP).2
Barotrauma
Barotrauma is the rupture of alveolar units, respiratory bronchioles, or bullae, causing extrapulmonary air to accumulate; it may be seen as pulmonary interstitial emphysema, pneumomediastinum, or pneumothorax. Pneumothorax in patients receiving positive pressure ventilation is often under tension and can have significant effects on hemodynamics. Barotrauma is more likely in patients ventilated with high airway pressures. However, it is the transalveolar pressure that determines the risk for barotrauma, not the airway pressure. As can be seen in Figure 29-1, transalveolar pressure is higher for the same airway pressure with reduced lung compliance than it is with reduced chest wall compliance or increased airway resistance.
Ventilator-Induced Lung Injury
In certain circumstances, positive pressure ventilation can cause or contribute to acute lung injury.3 Patients most at risk for ventilator-induced lung injury are those with severe systemic (e.g., sepsis) or pulmonary (e.g., pneumonia) disease who are ventilated with large tidal volumes, high airway pressures, inadequate levels of PEEP, and high inspired oxygen concentrations.4 The mechanisms of ventilator-induced lung injury are complex but involve an exacerbation of systemic inflammation5 and physical trauma to the alveoli due to overdistension and the repeated opening and closing of lung units.6 Crude mortality rate in critically ill patients ventilated with high tidal volumes (12 ml/kg) is 9% higher than the rate in those ventilated with low tidal volumes (6 ml/kg).7 This observation has led to the concept of lung-protective ventilation, which is described subsequently.
Cardiac Effects
The main direct effects of positive ventilation on the cardiovascular system are reduced systemic venous return,8 altered right ventricular afterload,9 and reduced left ventricular afterload.10 The impact of these effects is determined by the particular patient’s underlying cardiac function and intravascular volume status. Positive pressure ventilation performed properly eliminates or substantially reduces the work of breathing, which also has an indirect positive effect on cardiovascular function.
Right Ventricular Afterload
The effect of invasive ventilation on right ventricular afterload is variable and depends on lung volume. With low lung volumes (e.g., due to atelectasis) positive pressure ventilation and PEEP optimize lung volume and improve gas exchange, both of which reduce right ventricular afterload. With high lung volumes (due to excessive ventilatory pressures or high levels of PEEP) the pulmonary capillaries become compressed, which increases afterload.11 Changes in right ventricular afterload have a substantial impact on cardiac output in patients who are hypovolemic or who have right ventricular dysfunction.
Left Ventricular Afterload
Positive pressure ventilation increases intrathoracic pressure, which reduces the transmural pressure gradient across the left ventricle. This reduces systolic wall stress and therefore afterload (see Chapter 1; see Equation 1-3). Positive pressure ventilation can lead to a substantial improvement in cardiac output in patients with left ventricular dysfunction and is an important adjuvant for treating acute heart failure.
VENTILATOR MODES AND CONTROLS
Ventilators have become very complex and have a bewildering array of modes and controls,13 despite a lack of evidence of improved outcomes.14 Further confusion is created because manufacturers call similar ventilatory modes different names.
At its most simple, invasive ventilation involves mandatory machine-delivered breaths of a given volume (or pressure), in which controls for fractional inspired oxygen (FIo2), breath rate, breath volume (or pressure), and the ratio of inspiratory time to expiratory time (the I:E ratio) are set.15 For this type of mandatory ventilation to succeed, the patient’s respiratory system must be entirely passive. Much of the complexity of modern ventilators comes from the desire to allow patients to take spontaneous breaths that are assisted by and synchronized with the ventilator.
Inspiratory Flow Generation: Volume Control or Pressure Control
Volume control ventilation provides a constant inspiratory flow to achieve a predefined tidal volume (Fig. 29-2). Airway pressure varies with inspiratory time and changes in lung compliance and airway resistance. When setting the ventilator in volume control mode, a tidal volume of 6 to 8 ml/kg should be chosen.
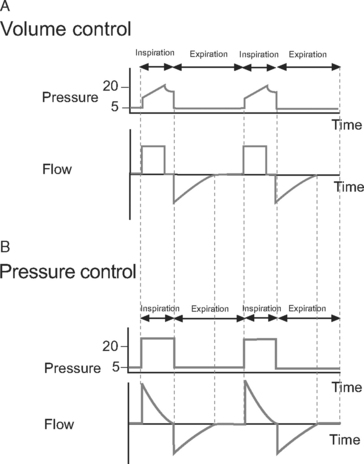
Figure 29.2 Pressure-time and flow-time curves for volume control and pressure control ventilation. A, Two volume control breaths are illustrated. The I:E ratio is approximately 1:2 and there is a short inspiratory pause at the end of inspiration. Plateau pressure is a slightly lower than peak pressure. Inspiratory flow is constant, whereas flow decreases during expiration. B, Two pressure control breaths are illustrated. The I:E ratio is approximately 1:2. No end-inspiratory pause is set. Inspiratory flow is decelerating and has fallen to zero by end-inspiration. In both A and B, expiratory flow has fallen to zero before the end-expiration. For the sake of clarity, the inspiratory rise time (see Fig. 28-4) has been set to zero; that is, peak inspiratory flow occurs immediately at the beginning of inspiration.
Pressure control ventilation provides a constant inspiratory pressure, which generates a decelerating inspiratory flow pattern (see Fig. 29-2). Tidal volume varies with inspiratory time and changes in lung compliance and airway resistance. The decelerating flow pattern distributes ventilation more evenly throughout the lung; in patients with V/Q mismatch, this improves oxygenation. It also allows some compensation for air leaks and limits inspiratory pressures, which helps to prevent ventilator-induced lung injury and barotrauma. The inspiratory pressure is chosen to achieve the desired tidal volume, usually 6 to 8 ml/kg, which typically requires a pressure of 15 to 30 cm H2O.16
Cycling
Time
In patients with increased airway resistance, a reduced respiratory rate and prolonged expiratory time is required to avoid dynamic lung hyperinflation (see subsequent material).17 For instance, a breath rate of 6 to 8 per minute with an inspiratory time of 1.3 seconds provides an expiratory time of 6.2 to 8.7 seconds and an I:E ratio of 1:4.7 to 1:6.7, respectively.
In patients with severe hypoxemia, a prolonged inspiratory time may enhance oxygenation by improving the ventilation of lung units with long time constants (see Chapter 1). In some circumstances, inverse ratio ventilation (e.g., an I:E ratio of 2:1 or 3:1) may be used (see Ventilatory Strategies in subsequent material).18
Flow
For some assisted modes of ventilation, such as pressure support, flow cycling is used to terminate inspiration and commence expiration. Termination of inspiration occurs when flow drops to a percentage of peak inspiratory flow (typically 0 to 25%). Flow cycling may improve patient-ventilator synchrony.19
Triggering
Triggering is the mechanism by which a patient’s spontaneous inspiratory effort is sensed and the ventilator is triggered to deliver inspiratory assistance. Thus, triggering is a method of cycling from expiration to inspiration that is not time dependent. Triggering is accomplished when the ventilator senses a change in flow or pressure within the ventilator circuit. Flow triggering is generally more sensitive than pressure triggering and results in improved patient-ventilator synchrony.15
Positive End-Expiratory Pressure
PEEP is positive pressure within the alveoli at endexpiration. It is applied by placing a pressure-limiting valve on the expiratory limb of the ventilator circuit. PEEP reduces extravascular lung water, prevents the opening and closing of small airways, and helps to recruit collapsed alveoli. This improves oxygenation, increases lung compliance, and reduces the risk of developing ventilator-induced lung injury.20,21 A low level of PEEP (5 cm H2O) should be applied to all patients who are invasively ventilated. High levels of PEEP (15 to 20 cm H2O) may be required in patients with acute respiratory distress syndrome (ARDS).22 Patients with increased airways resistance whose ventilators are set to an inadequate expiratory time may trap gas and generate auto-PEEP. By applying external PEEP at a level similar to that of the generated auto-PEEP, the inspiratory threshold load (see Fig. 27-4), and therefore the work of breathing, is reduced.
Ventilator Pressures and Graphics
Measured Pressures
Peak Pressure and Plateau Pressure.
Peak pressure is the maximum inspiratory pressure within the breathing circuit. It is affected by both respiratory compliance and airway resistance. Plateau pressure is the pressure within the breathing circuit following an end-inspiratory pause, which allows equalization of any pressure difference between the alveoli and the circuit. Thus, plateau pressure is indicative of alveolar pressure at end inspiration and is influenced by respiratory compliance alone. Plateau pressure may be measured (the patient must be heavily sedated, paralyzed, or both) using the inspiratory hold function on the ventilator or, in volume control mode, estimating it from the pressure-time curve (see Fig. 29-3) when an inspiratory pause or hold is used. Plateau pressure (PP) may be calculated from the respiratory system compliance (C) and the tidal volume (Vt), given:
Ventilator-induced lung injury is minimized by limiting plateau pressure to less than 32 cm H2O (and peak airway pressure to less than 35 cm H2O).4 However, when chest wall compliance is reduced (e.g., in cases of obesity or abdominal compartment syndrome), a higher plateau pressure may be tolerated (see Fig. 29-1).
With volume control ventilation, high peak and plateau pressures may be due to reduced lung or chest wall compliance (see Figs. 29-1 and 29-3), whereas high peak but normal plateau pressure is indicative of increased airway resistance (see Figs. 29-1 and 29-3).
End-Expiratory Pause Pressure and Auto-PEEP.
End-expiratory pause pressure is the pressure within the circuit following an end-expiratory pause, and it should equal the level of applied PEEP. If end-expiratory pause pressure is greater than the applied PEEP, auto-PEEP exists (Fig. 29-4).23 Auto-PEEP is caused by continued (delayed) emptying of alveoli at end-expiration and indicates increased airway resistance. The presence of auto-PEEP is suggested by continued gas flow at end-expiration on the flow-time curve (see Fig. 29-4).
Graphic Displays
Most ventilators can display real-time plots of derived respiratory indexes, which may be of assistance in adjusting ventilator settings.24 The most widely used are pressure-time and flow-time curves, dynamic pressure-volume loops, and flow-volume loops.
Pressure-Time and Flow-Time Curves.
Pressure-time and flow-time curves (see Figs. 29-2 to 29-4) are referred to in the earlier sections on flow generation, end-inspiratory pressure, and end-expiratory pressure. They are helpful in diagnosing abnormalities in compliance, resistance, and the presence of auto-PEEP.
The flow-time curve identifies whether flow has fallen to zero at end-expiration and end-inspiration. Continued flow at end-expiration indicates the presence of auto-PEEP (see Fig. 29-4). This may be remedied by increasing the expiratory time, which is usually achieved by reducing the breath rate. Continued flow at end-inspiration with pressure-control ventilation (see Fig. 29-3) may indicate increased airway resistance. It may be remedied by increasing the inspiratory time. However, if auto-PEEP exists as well, this may not be possible, and higher airway pressures may be required.
Dynamic Pressure-Volume Loops.
Dynamic pressure-volume loops (Fig. 29-5) provide a graphic representation of airway pressure against volume in real time for each breath. A dynamic pressure-volume loop differs from a static compliance curve because, in addition to the effect of respiratory system compliance, the effects of airway resistance, circuit resistance, and airflow are included.25
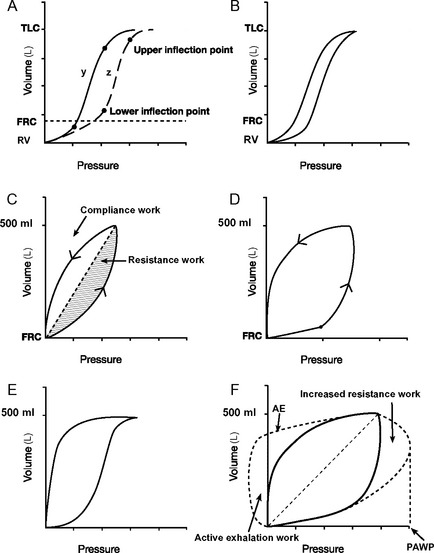
Figure 29.5 Pressure-volume loops. A, Two static pressure-volume curves (y and z; see also Fig. 1-15). Lower and upper inflexion points are shown on both curves. Above and below these inflexion points, compliance (ΔV/ΔP, the slope of the curve) is reduced. The lower inflexion point represents the lung volume at which some alveoli’s airways close (closing capacity); the upper inflexion point represents the start of overdistension. In curve y, the lower inflexion point lies below the functional residual capacity (FRC) and no airway closure occurs. In curve z, the inflexion point lies above the FRC, and airway closure will occur unless the FRC is increased by the application of PEEP. B, A dynamic pressure-volume curve is obtained by ventilating the lungs from residual volume (RV) to total lung capacity (TLC) when airways resistance is trivial. Hysteresis, in which lung volumes are different for a given pressure during inspiration and expiration is shown. Hysteresis is partly (but not solely) due to airway resistance. C, A dynamic pressure-volume curve is obtained when lungs are ventilated with a normal tidal volume from the FRC and airway resistance is normal. No inflexion points are seen, indicating no airway closure or overdistension. The shaded areas represent work to overcome respiratory system compliance (vertical hatch) and airway resistance (horizontal hatch). D, A dynamic pressure-volume curve with a lower inflexion point is indicative of inadequate PEEP. If a static pressure-volume curve were obtained, the FRC would be below the lower inflection point (curve z, A). E, A dynamic pressure-volume curve with an upper inflexion point is indicative of overinflation. This is termed “beaking.” F, The effect on the dynamic pressure-volume curve of increased airway resistance. Peak airway pressure (PAWP) and work of breathing (hatched areas) are increased because of the increased airway resistance. If expiration is active, the expiratory part of the curve will resemble the line labeled AE. Work done during active expiration is represented diagrammatically by the area labeled active exhalation work.
On a static respiratory system compliance curve (see Figs. 1-15 and 29-5A), upper and lower inflexion points can be identified. The upper inflexion point identifies overdistension of alveoli; the lower inflexion point identifies closing capacity. Ideally, the lungs should be ventilated between these two inflexion points, on the steep part of their compliance curves; that is, FRC should be above the lower inflexion point (as shown in Fig. 29-5A, curve y). The construction of a static compliance curve is impractical for routine care, and dynamic compliance curves are used instead (see Fig. 29-5B and C). Some ventilators can create an approximation of a static compliance curve by using the dynamic compliance curves generated by breaths of differing tidal volumes.
Inflexion points may be visible on dynamic-pressure volume curves but often they are not obvious. The presence of a lower inflexion point (see Fig. 29-5D) may indicate insufficient PEEP, whereas the presence of an upper inflexion point (see Fig. 29-5E
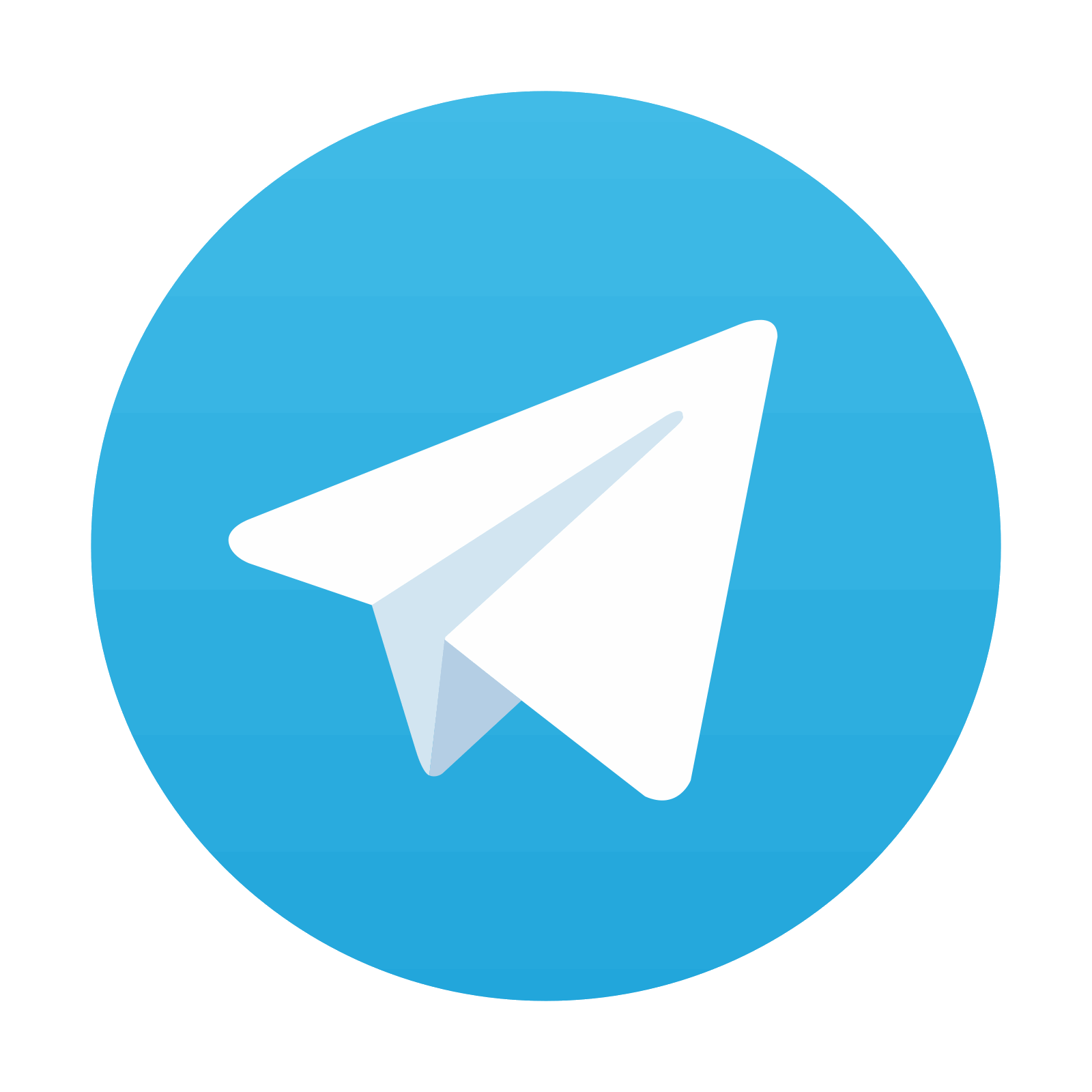
Stay updated, free articles. Join our Telegram channel
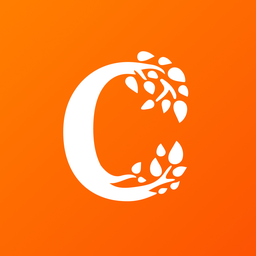
Full access? Get Clinical Tree
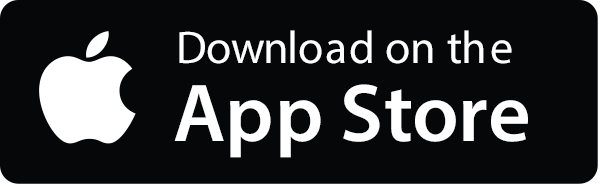
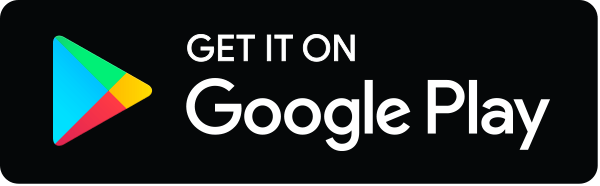
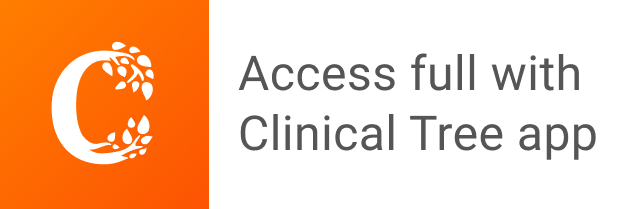