Abstract:
Invasive hemodynamics are fundamental to the understanding of cardiovascular physiology and pathophysiology. While many patients can be diagnosed and managed with noninvasive studies alone, invasive, catheter-based measurements performed in the cardiac catheterization laboratory represent the gold standard for hemodynamic evaluations. Such evaluations require meticulous attention to detail to maintain such standards. Functional information comes primarily from the recording of hemodynamic data of pressure measurements, cardiac output (CO), and blood oximetry.
Keywords:
invasive, hemodynamics, valvular, heart failure, pulmonary
Pressure waves in the heart
Blood within the heart and vessels exerts pressure. A pressure wave is created by cardiac muscular contraction and is transmitted from the heart chambers through the vessels. This wave can be measured by a catheter, which enables a closed fluid-filled column to be connected to a pressure transducer. The transducer in turn converts mechanical pressure to an electrical signal that is displayed on a video monitor.
Cardiac pressure waveforms are cyclical, with the pressure rising and falling from the onset of one cardiac contraction (systole) to the onset of the next contraction. The complete description of cardiac physiology can be found elsewhere, but an examination of the cardiac cycle, electrocardiogram (ECG), and corresponding pressures ( Figs. 4.1 and 4.2 ) provides a starting point to understand basic hemodynamics in the cardiac catheterization laboratory.



Collection of hemodynamic data is an integral part of every catheterization protocol. Even complex hemodynamic data recording can be accomplished accurately and rapidly if an efficient method is consistently used in the laboratory. A measurement sequence used in our laboratory is shown in Boxes 4.1, 4.2, and 4.3 . This sequence facilitates simultaneous pressure measurements across the heart. As with most brief techniques, it is not all-inclusive; different hemodynamic measurements for specific clinical situations are necessary. Specific examples are illustrated later.
The collection of routine hemodynamic data obtained from the right and left sides of the heart, with appropriate sampling of blood for oxygen saturations and CO measurements, can be accomplished in less than 30 minutes. Although the Fick CO method is considered to be more accurate, CO by the thermodilution (TD) technique is the routine. Depending on the clinical scenario, both methods of CO determination have their advantages and limitations. Arterial, vena caval, right atrial (RA), and pulmonary artery (PA) blood for oxygen saturation measurements are collected to screen for and, if identified, quantitation of intracardiac shunts.
Right- and left-sided heart catheterization
The protocol used during right-sided heart catheterization is summarized in Box 4.1 . Right-sided heart catheterization is performed for specific indications, most commonly in patients with a history of dyspnea, valvular heart disease, or intracardiac shunts ( Box 4.4 ). Patients with a history of pulmonary edema that occurred on a previous hospital admission often have only dyspnea with no objective evidence (e.g., after chest film, echocardiography) of left ventricular (LV) dysfunction. Dyspnea caused by lung disease cannot be differentiated from that caused by pulmonary hypertension or LV dysfunction. Right-heart catheterization (RHC) is most commonly performed with a pulmonary artery catheter (PAC), although larger-bore end-hole catheters may be used and provide high-fidelity tracings. First designed in the 1970s by Swan and Ganz, the PAC is available in sizes ranging from 5 F to 7 F and contains four ports: distal, proximal, thermistor, and balloon inflation. Additional ports for right ventricular (RV) pressure measurement, ventricular pacing, or drug infusion are available. The PAC may be inserted via femoral, subclavian, internal jugular, or basilic veins. Potential complications associated with RHC are shown in Box 4.5 .
Right atrium
- 1.
Advance catheter to inferior vena cava (IVC).
- 2.
Obtain oxygen saturation sample (1-mL heparinized syringe). a
- 3.
Advance catheter to superior vena cava (SVC).
- 4.
Obtain oxygen saturation sample (1-mL heparinized syringe). a
- 5.
Advance catheter to right atrium (RA).
- 6.
Record phasic and mean pressure (0- to 40-mm Hg scale, 25-mm/s sweep speed).
Right ventricle
- 1.
Advance catheter to right ventricle (RV).
- 2.
Record phasic pressure (0- to 40-mm Hg scale, 25-mm/s sweep speed).
Pulmonary artery and pulmonary capillary wedge
- 1.
Advance catheter to pulmonary artery.
- 2.
Record phasic/mean/phasic pressure (25/10/25-mm/s sweep speed).
- 3.
Obtain oxygen saturation sample (1-mL heparinized syringe). a
Flush catheter and advance to pulmonary capillary wedge (PCW).
Obtain oxygen saturation sample to confirm wedge position.
- 4.
Record phasic/mean/phasic pressure (25/10/25-mm/s sweep speed).
- 5.
Obtain oxygen saturation samples for arterial.
Perform Thermodilution Cardiac Output
a Only one to two drops of heparin should be aspirated and flushed out of 1- to 3-mL heparinized syringes.
Indications
Differentiation of shock (cardiogenic, distributive, hypovolemic, or obstructive)
Complications associated with acute myocardial infarction (i.e., hypotension, pulmonary edema, mitral regurgitation, ventricular septal defect, right ventricular (RV) ischemia, or tamponade)
Heart failure with reduced or preserved ejection fraction (diagnosis and management)
Primary and secondary pulmonary hypertension (diagnosis and management)
Valvular heart disease
Cardiac tamponade
Intracardiac shunts
Candidacy evaluation for orthotopic heart transplantation (OHTx)
Left ventricular (LV) assist device dysfunction
Acute pulmonary embolism
Assessing volume status in renal or hepatic failure
Postoperative monitoring after cardiac surgery
Primary lung disease
Contraindications (relative)
Prosthetic tricuspid or pulmonic valve
Coagulopathy
Severe thrombocytopenia
Endocardial pacemaker
Ventricular arrhythmias
Left bundle-branch block
Arrhythmias (atrial or ventricular)
Cardiac perforation
Pulmonary infarction
Pulmonary artery (PA) rupture
Air embolism
Endocarditis
Venous thrombosis
Arterial puncture
Pneumothorax
The most common complication of right-sided heart catheterization is arrhythmia resulting from mechanical catheter stimulation of the right ventricular outflow tract (RVOT), which can lead to ventricular tachycardia (VT), atrioventricular (AV) block or, rarely, right bundle-branch block. Significant but transient ventricular arrhythmias occur in 30% to 60% of RHC procedures and are self-limited (not requiring treatment). The arrhythmia is terminated when the catheter is readjusted. Sustained ventricular arrhythmias have been reported, especially in unstable patients or those with electrolyte imbalance, acidosis, or concurrent myocardial ischemia. In patients with left bundle-branch block, a temporary pacemaker may be necessary if right bundle-branch block occurs.
Proper catheter positioning is essential for proper waveform interpretation. Upon catheter insertion to 20 to 25 cm, a normal RA pressure tracing includes five components: (1) a wave of atrial contraction, (2) x descent illustrating atrial diastole, (3) c wave of tricuspid valve closure, (4) v wave of ventricular systole and passive atrial filling, and (5) y descent of passive atrial emptying (remember as a/x, c, v/y).
Advancing the PAC to 30 to 35 cm yields a RV waveform that can be identified by an abrupt increase in systolic pressure and a diastolic pressure that normally approximates the RA pressure. Rotation of the catheter through the RVOT and advancing across the pulmonic valve at 45 to 55 cm results in a PA waveform identified by three hallmark findings: (1) abrupt increase in diastolic pressure from RV to PA, (2) development of a small dicrotic notch in the PA tracing, and (3) alignment of the peak PA systolic pressure within the electrocardiographic T wave. Further advancement of the PAC results in occlusion of a secondary or tertiary branch of a PA and generates the pulmonary capillary wedge pressure (PCWP) waveform, characterized by a and v waves of reflected left atrial (LA) and LV contraction, respectively.
Use of pulmonary balloon occlusion (wedge) pressure
The PCWP closely approximates the LA pressure, reflecting the filling pressure of the LV in the in most patients. PCWP is not a reliable surrogate for LA pressure in patients with absence of mitral stenosis, cor triatriatum, or pulmonary veno-occlusive disease (e.g., pulmonary vein [PV] stenosis after atrial fibrillation [AF] ablation) ( Fig. 4.3 A). Reported discrepancies between LA pressure and PCWP may be caused in part by different types of catheters: Balloon-tipped flotation catheters are soft with small lumens, and transseptal pressure catheters (e.g., Brockenbrough or Mullins-type sheath) are stiff with large lumens. Transseptal LA catheterization should be considered when there is concern for these discrepancies.



Rules for obtaining an accurate PCWP that agrees with LA pressure are:
- 1.
Position the catheters correctly and verify position through waveform, oximetry (oxygen saturation >95%), and fluoroscopy. The wedged position of the catheter is confirmed by an oxygen saturation sample >95%. Note: Obtaining this saturation uncontaminated by low-saturation PA blood can be challenging because of the volume of low-saturation blood that must be discarded before wedge blood is collected. Use of a large-bore catheter and saline flushing during antegrade movement into the pulmonary capillary wedge (PCW) position can help with obtaining accurate oxygen saturation measurements.
- 2.
Confirm that PCWP is not a damped PA pressure by using a precise a and v waveform timed against the ECG or LV pressure. Use a stiff, large-bore, end-hole catheter and connect it to the pressure manifold with stiff, short pressure tubing. The system should be thoroughly flushed and bubble free.
Fick principle for measurement of cardiac output
The Fick principle states that uptake or release of a substance by any organ is the product of the arteriovenous concentration difference of the substance and blood flow to that organ. Pulmonary blood flow (which is equal to systemic blood flow in the absence of an intracardiac shunt) is determined by measuring the arteriovenous difference of oxygen across the lungs and the uptake of oxygen from room air by the lungs. CO is calculated as oxygen consumption divided by the arteriovenous oxygen (AVO 2 ) concentration difference. The AVO 2 consumption difference is calculated (in milliliters of oxygen) as the difference in left ventricular oxygen (LVO 2 ) content (1.36 × hemoglobin × LVO 2 saturation × 10) minus the pulmonary arterial (mixed venous) oxygen (PAO 2 ) content (1.36 × hemoglobin × PAO 2 saturation × 10).
With accurately measured oxygen consumption, the Fick technique is the most accurate method of assessing CO, particularly in patients with low CO. Supplemental oxygen is often administered to a patient during diagnostic cardiac catheterization. Mixing the supplemental oxygen with room air makes determination of the oxygen content of the inspired air difficult (if not impossible) to calculate. Supplemental oxygen therapy should be discontinued at least 10 to 15 minutes before determination of CO by the Fick technique.
There are several formulae used to compute assumed oxygen consumption (VO 2 ). Studies have shown that measured VO 2 differed significantly using values derived from formulae of Dehmer, LaFarge, and Bergstra ( Box 4.6 ) with median absolute differences of 28, 38, and 32 mL/min, respectively ( P <0.0001 for each). The measured and estimated values differed by >25% in 17% to 25% of patients, depending on the formula used. Median absolute differences were greater in severely obese patients (body mass index >40 kg/m 2 ) but were not affected by sex or age.
- 1.
Dehmer formula:
O 2 (mL/min) = 125 × BSA
- 2.
LaFarge formula:
O 2 (mL/min) = 138.1 − (11.49 × log age) + (0.378 × HR) × BSA (male);
O 2 (mL/min) = 138.1 − (17.04 × log age) + (0.378 × HR) × BSA (female)
- 3.
Bergstra formula:
O 2 (mL/min) = 157.3 × BSA + 10 − (10.5 × log age) + 4.8 (male);
O 2 (mL/min) = 157.3 × BSA − (10.5 × log age) + 4.8 (female)
BSA, Body surface area; HR, heart rate; O 2 , assumed oxygen consumption.
Compared with measured oxygen consumption, the assumed Fick at 3 mL O 2 /kg correlated poorly. This assumption using the LaFarge equation is based on pediatric measurements and is specifically not recommended for use in adults, despite the fact that it is common practice. The most accurate method is direct measurement of VO 2 at the time of PA and arterial blood sampling and can be facilitated by metabolic carts (e.g., MCG Diagnostics) (see Fig. 4.3 B). These direct measurements of VO 2 also enable cardiac function assessment with cardiopulmonary exercise testing. Estimates of resting VO 2 derived from conventional formulae are inaccurate, especially in severely obese individuals. When accurate hemodynamic assessment is important for clinical decision-making ( Box 4.7 ), VO 2 should be directly measured.
- 1.
Aortic Stenosis and/or Regurgitation
- a.
Poor Doppler signature by echocardiography
- b.
Discrepancy between symptoms and noninvasive testing
- c.
Severe anemia
- d.
Concomitant subvalvular/supravalvular stenosis
- e.
Low-output/low-gradient AS with angiographic assessment for concomitant coronary disease before dobutamine challenge
- f.
Low-output/low-gradient AS with preserved left ventricular (LV) ejection fraction; vasodilator challenge if high peripheral vascular resistance
- a.
- 2.
Mitral stenosis and/or regurgitation
- a.
Discrepancy between symptoms and transmitral gradient or pulmonary pressures by noninvasive testing
- a.
- 3.
Pulmonary hypertension (primary and secondary)
- 4.
Heart failure with preserved ejection fraction (HFpEF) without valvulopathy
- 5.
Candidacy evaluation for LV assist device or orthotopic heart transplantation (OHTx)
- 6.
Right ventricular (RV) failure
- 7.
Hypertrophic cardiomyopathy (HCM)
- 8.
Constrictive and/or restrictive cardiomyopathy
Indicator dilution cardiac output principle
The indicator dilution technique is based on the principle that a single injection of a known amount of an indicator (e.g., cold saline for the TD technique) injected into the central circulation mixes completely with blood and changes concentration as it flows to a more distal location. The change in the indicator concentration (or temperature) is plotted over time; the area under the curve is used to calculate CO.
Thermodilution indicator method
The TD indicator method requires a PA balloon flotation catheter (Swan-Ganz) with a thermistor at the tip. The TD Swan-Ganz catheter is a triple lumen design. The proximal port, located 30 cm from the tip, is used for RA pressure measurement and rapid infusion of the saline indicator during CO determination, the distal end-hole is used for pressure measurement, and the lumen is used to inflate the balloon. A thermistor at the distal tip measures blood temperature.
The balloon serves two purposes: (1) a positioning aid and (2) facilitation of PCWP. The inflated balloon helps to direct the catheter into the PA by “floating” with the flow of blood through the right-heart chambers. When positioned in the PA, the catheter can be advanced to the distal pulmonary vasculature, “wedging” in a small branch. The balloon forms a seal that isolates the tip from PA flow to measure LA pressure. The thermistor at the end of the catheter is positioned in the PA when the proximal port is in the RA.
Measuring cardiac output
CO is determined by rapid-injection 10 mL of iced (4°C) or room temperature (20°C) saline injected as the indicator through the proximal port of the PAC. An external thermistor measures the temperature of the saline injectate. Complete mixing of the injectate with blood causes a decrease in blood temperature, detected by the distal thermistor. The CO computer calculates the change in indicator concentration (temperature over time) to determine CO in liters per minute. To obtain consistent, reliable results, the physician and technical staff must use appropriate technique. The following steps contribute to TD CO accuracy.
- 1.
Position the catheter properly in the PA. Excessive coiling of the catheter in the RA or RV can result in poor positioning of the dual thermistor in relation to the injectate port. This problem is sometimes encountered with a large, dilated right side of the heart. In these instances, a 0.025-inch guidewire can be used to give additional stiffness to the catheter. A wedged catheter does not register the appropriate temperature change.
- 2.
Most TD systems require input of a specific computation constant (in the output computer) depending on the system used. Some computers provide a table that gives a constant corresponding to the injectate volume and temperature and size and type of catheter used.
- 3.
Deliver the precise amount of injectate, which is relatively cool in temperature relative to the patient. In adults, 10 mL is the commonly used volume, and 5 mL is typical in children. In adults for whom fluid restriction is important, a smaller bolus of injectate may be desired. If so, remember to change the computation constant on the output computer to reflect the change in injectate volume.
- 4.
Coordinate the start button and bolus injection. Press the start button on the computer followed within a few seconds by the bolus injection. Injection before the release of the start button is a common error. When this happens, the computer does not recognize the full bolus of injectate delivered.
- 5.
Deliver the bolus rapidly at a constant flow rate. Use two hands to inject.
- 6.
Securely connect the catheter to the computer. The interface cable from the CO computer is nonsterile so care should be taken not to contaminate the catheterization table.
- 7.
If room temperature saline is used, there should be at least a 10°C difference between the injectate and body temperature. Most computers have built-in sensors that enable the technician to check these parameters before the start of the procedure.
- 8.
Obtain three to five CO values. Erroneous values are ignored in the final averaging of results.
- 9.
Keep in mind that TD technique is inaccurate with tricuspid regurgitation or low CO or significant arrhythmia, which may occur from ectopy caused by placement of the catheter. Both conditions interfere with the normal flow of injectate past the sensing thermistor. Intracardiac shunts further reduce the accuracy of TD.
Left-sided heart catheterization
The protocol for left-sided heart catheterization is summarized in Box 4.2 and indications for left-sided heart catheterization are summarized in Chapter 1 . A combined right-side and left-side heart protocol is a precise and complete method for addressing the most common hemodynamic problems in the catheterization laboratory (see Box 4.3 ).
Aortic valve assessment
- 1.
Match peripheral to central aortic (Ao) pressure. If using pigtail catheter inserted through arterial sheath (sheath should be 1 F larger than catheter). If using double lumen catheter, match two Ao pressures before crossing valve.
- 2.
Administer heparin (40 U/kg per laboratory routine).
- 3.
Advance wire and catheter across Ao valve.
- 4.
Obtain zero left ventricular (LV) pressure.
- 5.
Obtain zero sheath/Ao pressure.
- 6.
Record sheath/Ao pressure and LV pressure simultaneously (0- to 200-mm Hg scale).
* Right-sided heart hemodynamic studies often precede left-sided heart studies. Simultaneous pressures of the left and right sides of the heart provide the most precise and accurate information.
- 1.
Perform right-sided-heart catheterization and position catheter in pulmonary capillary wedge (PCW).
- 2.
Advance left-heart catheter to left ventricle (LV).
- 3.
Aortic (Ao) valve assessment: Follow left-sided heart protocol (see Table 4.2 ).
Table 4.2
Oxygen Saturation Values for Shunt Detection.
Level of Shunt
Significant Step-Up Difference a O 2 % Saturation
Atrial (SVC/IVC to right aorta)
≥ 7
Ventricular
≥ 5
Great vessel
≥ 5
IVC, Inferior vena cava; SVC, superior vena cava.
MVO 2 = (3 SVC + 1 IVC)/4 and difference from PA should normally be ≤7%.
a Difference between distal and proximal chamber; for example, for atrial septal defect (ASD).
- 4.
Mitral valve assessment:
Obtain zero PCW and femoral arterial (FA) and LV pressures.
Record LV vs. PCW (50-mm/s speed, 0- to 40-mm Hg scale).
Let down balloon or pull back PCW to pulmonary artery (PA) (40-mm Hg scale).
Note: If mitral valve gradient is present, 100-mm/s sweep.
Measure cardiac output (CO): Thermodilution (TD) outputs × 3.
Obtain arterial oxygen and pulmonary arterial oxygen (PAO 2 ) saturation samples.
- 5.
Right-sided heart pull back:
Record PCW to PA (40-mm Hg scale).
Record PA to right ventricular (RV) pressure.
To assess constrictive/restrictive physiology, record RV and LV simultaneously (capture both 0-40 mm Hg and 0-200 mm Hg to observe dynamic RV/LV respiratory changes).
Record RV to RA (0- to 40-mm Hg scale).
Left ventriculography usually performed at this point.
- 6.
Postventriculography hemodynamics:
Record postventriculography left ventricular end-diastolic pressure (LVEDP) (0- to 40-mm Hg scale).
Perform LV pull back to aorta with sheath/Ao pressures displayed (0- to 200-mm Hg scale).
Computations for hemodynamic measurements
When hemodynamic data have been obtained, specific computations quantify cardiac function. In this section, some of the most common computations and standard formulae are provided for measurement of cardiac work, flow resistance, valve areas, and shunts. Complete derivations and applications of these formulae can be found elsewhere.
CO using the Fick principle and O 2 consumption is:

Oxygen consumption is best measured from a metabolic “hood.” (This device is widely used except in a pulmonary function laboratory.) It is more commonly estimated as 3 mL O 2 /kg or 125 mL/min/m 2 . The true O 2 consumption for an adult based on the preceding estimate has been strongly questioned. AVO 2 difference is calculated from arterial–mixed venous (PA) O 2 content, where O 2 content is saturation × 1.36 × hemoglobin.
For example, if arterial saturation is 95%, O 2 content 0.95 × 1.36 × 13.0 g = 16.7 mL, PA saturation 65%, O 2 consumption 210 mL/min (70 kg × 3 mL/kg) or measured value, CO is determined as follows:

- 7.
Transpulmonary gradient (TPG; mm Hg) is
TPG = mean pulmonary artery pressure (mPAP) − mean RA pressure
- 8.
PA capacitance (PAC: mL/mm Hg) is
PAC = stroke volume/PA pulse pressure
- 9.
Systemic vascular resistance (SVR; Wood units) is
where R is resistance; Δp is mean pressure differential across the vascular bed, and is blood flow. Resistance units (mm Hg/L/min) are also called hybrid resistance units or Wood units . To convert Wood units to metric resistance (dynes × s × cm − 5), multiply by 80.
Computations of valve areas from pressure gradients and cardiac output
A pressure gradient is the pressure difference across a valvular or vascular obstruction, such as a stenosis or narrowed valve. Figure 4.3 C is a diagram of a coronary stenosis with higher pressure proximal to the stenosis and lower pressure beyond the stenosis. The same principle applies to heart valves with higher pressure proximal to the stenotic valve and lower pressure distal to the stenotic valve. Several methods are used to measure transvalvular gradients.
Techniques to measure LV-aortic (Ao) pressure gradients are listed in Box 4.8 .
Single catheter left ventricular (LV)-aortic (Ao) pull back
LV and femoral sheath
LV and long Ao sheath
Bilateral femoral access
Double lumen pigtail catheter
Transseptal LV access with ascending Ao
Pressure guidewire with ascending Ao
Multitransducer micromanometer catheters
All pressure gradients are affected by a number of physiologic, anatomic, and artifactual variables ( Box 4.9 ). Physiologic variables include (1) rate of blood flow (e.g., CO, coronary blood flow), (2) resistance to flow, and (3) proximal chamber pressure and compliance. Anatomic variables include (1) shape and length of valve orifice; (2) tortuosities of the vessels (for arterial stenosis), folding of coronary artery by guidewire (i.e., pseudostenosis); and (3) multiple or serial lesions (for cardiac valves and arterial stenosis).
Miscalibrated pressure transducers
Pressure leaks on catheter manifold or connecting tubing
Pressure tubing type, length, and connectors
Air in system
Catheter sizes (especially those with small diameters)
Fluid viscosity (viscous contrast material tends to damp pressure wave)
Position of catheter side holes moving across aortic (Ao) valve (see Fig. 4.16 )
Fig. 4.16
A falsely wide aortic (Ao) pulse pressure (*) with the use of a pigtail catheter is caused by incomplete withdrawal of all side holes outside the left ventricle (LV) . On final catheter positioning (far right) , Ao pressure is normal. ECG, Electrocardiogram.
Valve area calculations

where MVG is mean valvular gradient (mm Hg), K (44.3) is a derived constant by Gorlin and Gorlin, C is an empirical constant that is 1 for semilunar valves and 0.85 for the mitral valve, and valve flow is measured in milliliters per second during the diastolic or systolic flow period.


where systolic ejection period (s/min) is systolic period (s/beat) × heart rate (HR).
Examples of aortic and mitral valve area calculations
Most modern physiologic recording systems use computer-generated waveforms and gradient-producing valve areas automatically. When using femoral artery (FA) and LV pressure, time shifting the FA back to match upstroke of the LV will underestimate the true Ao valve gradient ( Fig. 4.4 ). When calculating from the formula, convert CO to milliliters per minute, not liters per minute. When computing flow, convert the ejection period and filling period to fractions of the period in seconds.

Data obtained at catheterization for Ao stenosis ( Fig. 4.5 ) are:
- 1.
CO = 4 L/min = 4000 mL/min
- 2.
HR = 60 beats/min
- 3.
Pressure waves of LV-Ao pressures are displayed at 100-cm/s sweep speed. If FA pressure is used, greater accuracy is associated with unshifted Ao upstroke and LV pressure.
Step 1. Obtain the Ao-LV gradient area (if in AF, the average is 10 beats).

Notes on the aortic valve gradient
The mean pressure gradient (MPG) is the area of superimposed Ao and LV pressure tracings. Peak-to-peak pressure difference is easily measured and often used as an estimate of valve stenosis severity. Peak-to-peak pressure difference is not equivalent to the mean gradient for mild and moderate stenosis but is often close to the mean gradient for severe stenosis.
The delay in pressure transmission and pressure wave reflection from the proximal aorta to the FA artificially increases the mean gradient. If the pressure tracing is shifted to match the upstroke of the LV, femoral pressure overshoot (amplification) reduces the true gradient. In patients with low gradients (i.e., <35 mm Hg), more accurate valve areas were obtained with unshifted LV-Ao pressure tracings (see Fig. 4.4 A). Consider changing the default option on the computer to shift Ao pressure automatically to correspond with LV pressure (see Fig. 4.4 B). A dual lumen pigtail catheter will eliminate the delay in pressure transmission and femoral pressure amplification but the side arm must be meticulously flushed to avoid damping. Figure 4.5 shows LV-Ao pressure measurement from the central Ao catheter without delay in the upstroke of Ao pressure.
Simplified formulae provide quick in-laboratory determinations of AVA. AVA can be accurately estimated as CO divided by the square root of the LV-Ao peak-to-peak pressure difference.


Note: The quick formulae for valve area differ from the Gorlin formula by 18 ± 13% in patients with bradycardia (<65 beats/min) or tachycardia (>100 beats/min). The Gorlin equation at low-flow states overestimates the severity of valve stenosis.
Use of valve resistance for aortic stenosis
Although not commonly computed, valve resistance (a measure of valve obstruction) has been shown to have clinical value. Valve resistance has not been used because the units of dynes/s/cm −5 were not well related to clinical outcomes.
Valve resistance is calculated using the same variables for valve area measurement. In contrast to valve area, the MPG is considered to be a linear variable rather than taken as a square root term. The contribution of pressure gradient to the magnitude of valve resistance is greater. Resistance has also been shown to be more constant than valve area under conditions of changing CO. Figure 4.6 shows resistance and area calculated in a group of patients before and after balloon Ao valve dilation. Resistance rises sharply above a valve area of 0.7 cm 2 . The shoulder of this curve is 0.7 to 1.1 cm 2 , which is the common area of indeterminate significance of Gorlin AVA. Some patients in this gray zone tend to have higher valve resistance than others. In this setting, it has been shown that patients with resistance >250 dynes/s/cm −5 are more likely to have significant obstruction than patients with resistance <200 dynes/s/cm –5 . There is also a gray zone in using this index; some patients may have resistance <250 dynes/s/cm −5 despite a planar valve area of 0.7 to 0.8 cm 2 . Resistance is a complementary index, not a replacement for valve area.

Catheter selection for aortic stenosis
Initial catheter selection is a matter of operator choice and experience. In most cases, dual lumen pigtail ventriculography catheter is a good initial choice. Of note, the side port of the dual lumen catheter is prone to damping, should be flushed routinely, and must be carefully positioned to ensure its placement above the Ao valve in the central aorta. The Ao valve is usually crossed using a 0.038-inch straight-tipped guidewire loaded into a steerable catheter (e.g., AL-1 or Judkins right). Manipulation of the wire and catheter allows wire positioning in various directions to cross the valve. After wire crossing, advance the LV catheter over the wire and position correctly for ventriculography and hemodynamic studies. Only the pigtail catheter is suitable for LV angiography. It should not require more than several minutes to cross an Ao valve, even if severely stenosed. The wire should be removed every 3 minutes during an attempt to be wiped. If great difficulty is encountered with crossing, a transseptal approach should be considered.
Points to remember when crossing the aortic valve with guidewires
- 1.
Give adequate heparinization (a 40- to 50-U/kg bolus) anticipating 10 minutes of work. Perform frequent catheter flushing (approximately every 2 to 3 minutes) on removal of the guidewire.
- 2.
To prevent guidewire clotting, a maximum of 3 minutes per crossing attempt is a good rule before wire withdrawal, wiping, and flushing of the catheter.
- 3.
To prevent dissection of the coronary arteries, avoid positions of the catheter and guidewire that point to the coronary ostia. This is achieved most easily by using left anterior oblique views for crossing.
- 4.
Practice gentle manipulation of the wire to avoid damaging the valve, lifting atheromas, or causing a perforation of the cusps or Ao root.
- 5.
After valve crossing and during catheter exchanges, observe the distal guidewire position in the ventricle to avoid wire perforation. Once the catheter is in the ventricle, the tip of the exchange guidewire can be shaped in a curve to prevent perforation during further catheter exchanges.
When do you need to cross the aortic valve for hemodynamic assessment?
An example of a class-I recommendation is when experts recommend a procedure for direct hemodynamic measurement in symptomatic aortic stenosis (AS) patients for whom noninvasive tests are inconclusive or in whom a discrepancy exists between the noninvasive test and clinical findings regarding the severity of Ao stenosis (level C).
It is a class III recommendation (experts agree procedure provides no benefit or may be harmful) not to cross the Ao valve during cardiac catheterization when noninvasive tests are adequate and concordant with clinical findings (level C), or for the assessment of severity of Ao stenosis in asymptomatic patients (level C).
If the echocardiogram can provide precise, accurate, reproducible, high-quality, and high-confidence information, crossing of the Ao valve is relatively superfluous. This would also apply to any routine catheterization, because LV function can readily be assessed by echocardiography. However, in the patient with Ao stenosis in whom there is a question about the adequacy of the noninvasive testing, retrograde cannulation with catheter-obtained hemodynamics of the Ao valve is certainly important in determining the true transvalvular gradient because aortic valve replacement is indicated when the stenosis is severe in symptomatic patients. Thus, the decision of whether to cross the valve or not remains patient-specific.
Data from the catheterization laboratory for calculation of mitral valve area
Figure 4.7 shows a hemodynamic tracing to calculate the MVA.
- 1.
CO = 3.5 L/min = 3500 mL/min
- 2.
HR = 80 beats/min
- 3.
Scale factor = 1 cm = 3.9 mm Hg (40 mm Hg full scale)
- 4.
Tracing of LV-PCW pressure at 100-mm/s paper speed = (10-cm/s paper speed) (align PCW v wave with downstroke of LV pressure)
Step 1. Planimeter five LV-PCW areas (10 if in AF).
Area = 9.46 cm 2
Step 2. Measure diastolic filling period (DFP).

Step 4. Compute mitral valve flow. For the mitral gradient (similar to AVAs), DFP is in centimeters at this point, owing to scale factor.
Notes on mitral valve gradient
Obtaining an accurate PCWP is crucial (see earlier discussion about PCW accuracy; see Fig. 4.3 A). Use of direct LA pressure from transseptal measurement is the most accurate method. Transseptal catheterization will confirm pressure gradients, especially for suspected prosthetic mitral stenosis. However, if the PCWP-LV pressure tracings show no significant gradients, transseptal catheterization is unnecessary.
Simplified mitral valve gradient calculation by Cui et al.
Cui et al. (2007) simplified estimation of the mitral valve gradient and thus simplified the calculation of MVA from hemodynamic tracings. Because the mean mitral valve gradient is the pressure difference between the mean left atrium pressure (MLAP) and mean left ventricular pressure (MLVP) during diastole (i.e., MLVG = MLAP − MLVP), the computation of the mean mitral valve gradient depends on simply knowing the MLVP. The MLAP is easily obtained from the electronically meaned LA signal on the hemodynamic recorder. The area under the LV pressure during diastole is roughly a triangle with the three corners formed from the intersections of the DFP starting and ending points (mitral valve closure and opening) marking the vertical lines intersecting with the LV pressure line (rising diagonally across diastole; Fig. 4.8 ). This triangular area can be estimated from the rectangular area LVEDP × DFP divided by 2. Thus, the MLVP is equal to the LVEDP/2. From this key calculation, the mitral valve gradient is therefore simplified as


There is strong correspondence between MVAs calculated by the Gorlin and Hakki formulae, both before and after mitral balloon valvuloplasty, with an error of estimates at 1.6 mm Hg. The Cui mitral valve gradient slightly overestimated the gradient before but not after mitral valvuloplasty. The Hakki formula significantly underestimated mitral valve gradients after mitral balloon valvuloplasty. Unlike Hakki, the Cui mitral valve gradient was not affected by mitral regurgitation, Ao insufficiency, AF, or HR.
Although simple, the Cui mitral valve gradient still has potential problems. HR changes will affect the shape of the triangular area under the LV pressure curve, and tachycardia may cause a potential overestimation of valve severity.
Tricuspid valve gradients
Because small gradients (5 mm Hg) across the tricuspid valve may lead to significant clinical symptoms, precise measurement of hemodynamics through two large-lumen catheters may be required. Match pressures through two catheters (or through the two lumens of a balloon-tipped catheter if correctly positioned) before placement in the RA and RV to avoid technical error. The Gorlin valve area formula has not been validated for the tricuspid or pulmonic valves.
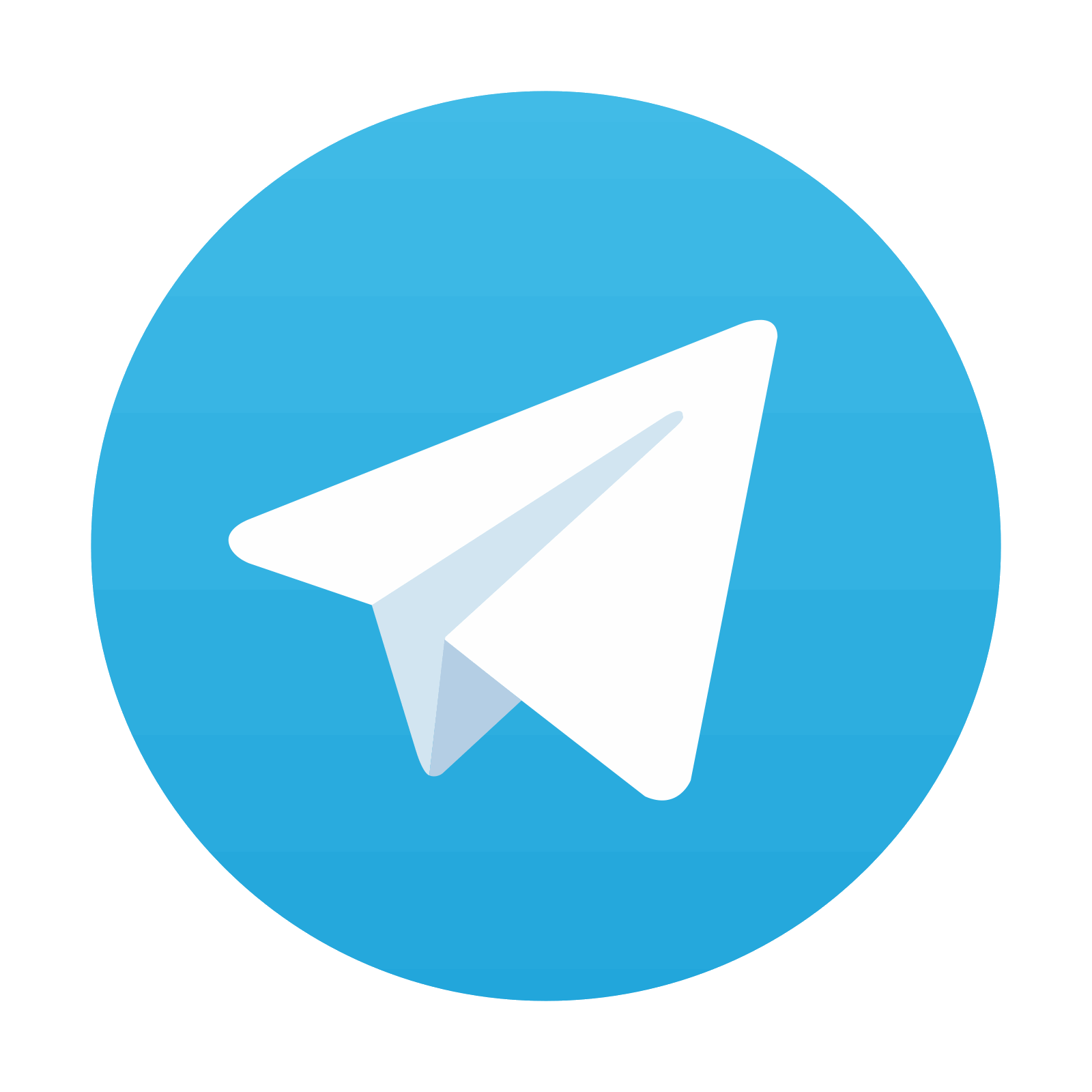
Stay updated, free articles. Join our Telegram channel
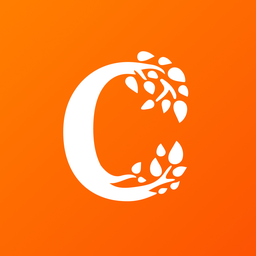
Full access? Get Clinical Tree
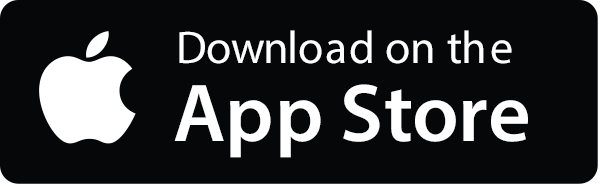
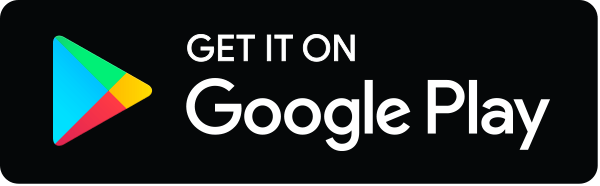