Fig. 1.1
Spatio-temporal patterns in different excitable media. a Chemical waves in the two-dimensional Belousov-Zhabotinsky reaction. Waves originating from the lower left corner have been broken by disturbing the solution in the petri dish with a pipette. Picture by C. Richter. b Spiral wave patterns in a colony of starving dictyostelium discoideum (difference between two consecutive images, picture by D. Loh and A. Bae). c Contraction waves in cultured cardiomyocytes from neonatal rats, made visible by difference imaging. Picture by C. Richter
1.1 Anatomy of the Heart
The heart is the central pumping organ for blood in all higher organisms and drives the circulatory system that is designed to supply all body tissues with adequate amounts of oxygen and nutrients and to remove carbon dioxide and other waste products. In humans, it is a four-chambered hollow muscle (see Fig. 1.2), which, by coordinated contraction, transports de-oxygenated blood to the lungs and oxygenated blood to other organs including the extremities of the body.1 The four chambers consist of two ventricles, each receiving fluid inflow from its adjacent atrium during one heart beat. A system of valves ensures that, during the sequence of contractions (systole) and relaxations (diastole) of the different chambers commonly referred to as the cardiac cycle, blood is taken in from the veins and pumped out through the arteries. The right heart (the right atrium and ventricle as a whole) is responsible for the pulmonary circulation, receiving oxygen-depleted blood from the body and releasing it through the right ventricle into the pulmonary arteries. After the lung has replenished the blood with oxygen, it enters the left heart through the left atrium and is pumped out through the heart’s biggest artery, the aorta, which is connected to the left ventricle. One of the amazing properties of the heart is that it also supplies blood to its own tissue: Just outside the aortic valve, the left and right coronary arteries branch off from the aorta. They run in between the pericardium, a protective sac for the heart, and the epicardium, the outermost layer of actual muscle tissue (myocardium). From there they branch off into the myocardium, splitting into smaller and smaller vessels which provide the necessary blood supply. A similar tree-like structure is formed by the coronary veins which remove oxygen-depleted blood and waste products from the muscle.
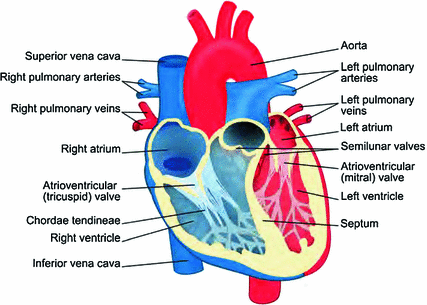
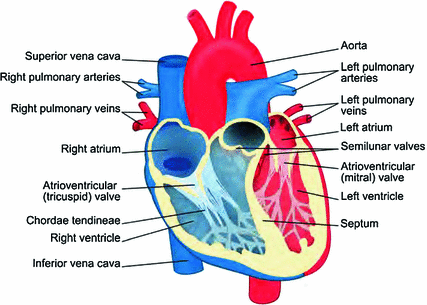
Fig. 1.2
Anatomy of the heart. Cavities and vessels transporting oxygenated blood are colored in red, those filled with oxygen-depleted blood are marked blue (“Modified version of picture published by user ZooFari as Heart diagram-en.svg at http://commons.wikimedia.org/wiki/File:Heart_diagram-en.svg under CC BY-SA 3.0: http://creativecommons.org/licenses/by-sa/3.0/deed.en; the modified image may be reused under the same conditions”)
The two atrioventricular valves separating the atria and the ventricles are connected via the chordae tendineae to the papillary muscles and trabeculae of the ventricles. The former are large-scale finger-shaped protrusions from the bulk of ventricular muscle tissue, while the latter are beam-like muscle strands, connected to the bulk tissue in different places. They contract together with the ventricles and thereby pull on the chordae tendineae, thus preventing valve prolapse into the atrium due to the high pressure during the ventricular systole.
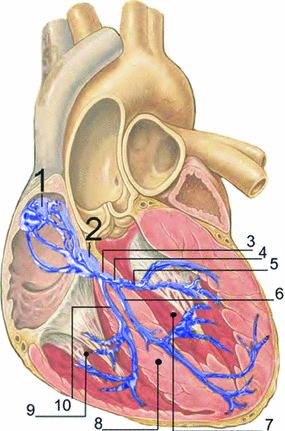
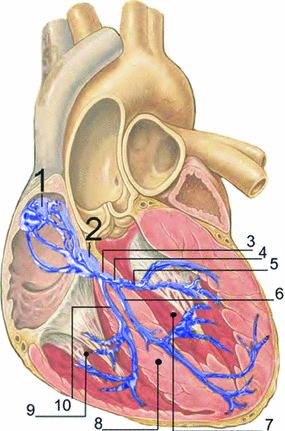
Fig. 1.3
Electrical conduction system of the heart. (1) Sinoatrial node; (2) atrioventricular node; (3) bundle of His; (4) left bundle branch; (5) left posterior fascicle; (6) left-anterior fascicle; (7) left ventricle; (8) ventricular septum; (9) right ventricle; (10) right bundle branch (“Picture published by user J. Heuser as RLS 12blauLeg.png at http://commons.wikimedia.org/wiki/File:RLS_12blauLeg.png under CC BY 2.5: http://creativecommons.org/licenses/by/2.5/ based on work by Patrick J. Lynch and C. Carl Jaffe, MD, Yale University School of Medicine, Center for Advanced Instructional Media, 1987–2000, see http://commons.wikimedia.org/wiki/File:Heart_anterior_view_coronal_section.jpg”)
1.2 Physiology of the Heart
The cardiac cycle is controlled by electrical signals traveling through the heart.2 The phenomenology of the heart’s bioelectricity has been known since the end of the nineteenth century, when time-varying potential differences on the body surface were discovered which are correlated with the heart beat. They were made practically available as electrocardiograms (ECGs) for diagnosis by W. Einthoven through his invention of the electrocardiograph, for which he received the Nobel Prize in 1924. On the microscopic scale, these electrical signals are known today to correspond to action potentials performed by individual muscle cells (cardiomyocytes), similar to those of neurons (with some important qualitative differences, see Sect. 1.2.1). The pathway for excitation from initiation to the contraction of the ventricles is shown in Fig. 1.3. In the sinoatrial node located in the right atrium, specialized pacemaker cells spontaneously generate action potentials, which, under normal physiological conditions, are considered the start of the cardiac electrical conduction system. Action potentials are rapid transient alterations of a cell’s transmembrane voltage. The rate at which the pacemaker cells depolarize defines the heart rate and is influenced by the autonomous nervous system. The sinoatrial node directly excites the muscle tissue of the right atrium from which the activity can propagate to the left atrium via different connections, the fastest of which is usually Bachmann’s bundle. Any activity spreading to the ventricles has to pass through the atrioventricular node located at the top of the tissue separating the two ventricular chambers of the heart, the septum. The atrioventricular node delays the conduction of the signal by about
, ensuring the ventricles are filled before contraction occurs. Additionally, it is the first in a cascade of (slower) backup pacemakers which can initiate contraction of the ventricles in case the input from the sinoatrial node is absent or blocked.3 From the atrioventricular node, the His bundle and the Purkinje fibers conduct action potentials down the septum and to the left and right ventricles. The fibers of the electrical conduction system consist of specialized cardiomyocytes and are connected to the actual contracting tissue only in a few places. Within the bulk of tissue, the activity propagates via gap junctions connecting individual cardiomyocytes to their neighbors. The arrangement of excitatory connections between different parts of the heart therefore coordinates the activation sequence, which is indispensable for the heart to effectively fulfill its function.

1.2.1 Cardiomyocytes
Like all cells, cardiomyocytes in their resting state have a finite membrane voltage or potential, which is defined as the electrical potential within the cell with respect to the extracellular space. It arises from the interaction of electrical and ion concentration gradients across the cell membrane. Inside the cell, the concentration of Na
, Ca
and Cl
ions is much lower than in the extracellular fluid, while the converse is true for K
ions. These different ion concentrations are maintained by active membrane proteins including the Na
/K
ATPase and Ca
ATPase, which derive their energy from the hydrolysis of adenosine triphosphate. The fact that, under normal physiological conditions, the ion concentrations are stable is called ion homeostasis.







For a single ion species, the difference in concentration across the membrane causes a directed net flow of ions that seeks to compensate the concentration gradient, provided the membrane has a non-zero permeability. However, since the diffusing particles are charged, this leads to the buildup of an electrical gradient, counteracting the concentration gradient. The electrical potential difference between the intracellular and the extracellular space, when these two opposing gradients balance, is called the reversal potential of the ion species. It can be calculated from the Nernst equation (see Ref. [19], pp. 78–83)
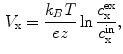
where
refers to the ion species,
and
are its extracellular and intracellular concentrations, respectively,
is Boltzmann’s constant,
is the elementary charge and
is the degree of ionization of the ion species. Since the ion concentrations are more or less constant due to ion homeostasis, each ion species has a fixed reversal potential. Using Eq. (1.1), these evaluate to approximately
for K
,
for Na
,
for Cl
and
for Ca
.
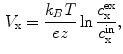
(1.1)














When multiple permeating ion species are present, the resulting resting potential can be calculated using the Goldman-Hodgkin-Katz equation [20, 21], in which the concentrations
of Eq. (1.1) are replaced by weighted sums for the different ion species and the weights are given by the corresponding membrane permeabilities.4 Specialized pores in the cell membrane, so-called ion channels, selectively determine the permeability of the membrane for different ion species. At rest, the membrane is mainly permeable to K
, which is why the resting membrane potential of a cardiac cell is between
and
.
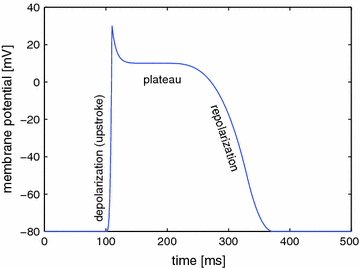




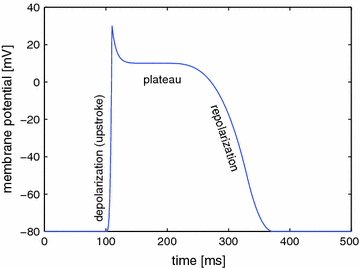
Fig. 1.4
Cardiac action potential
The permeability of the membrane for individual ion species can change rapidly, because ion channels are active elements that can open and close in response to external stimuli. This mechanism of ion channel gating is what gives the membrane potential its dynamics and the cell the ability to perform action potentials. A schematic sketch of the cardiac action potential is shown in Fig. 1.4. A small depolarization of the cell above a critical threshold between
and
increases the opening probability of voltage-gated sodium channels and initiates a positive feedback process: the increased permeability drives the membrane potential more towards the reversal potential of sodium, which leads to the opening of even more sodium channels. Since the Na
ions move down both the electrical and concentration gradient, this process results in a rapid upstroke with an amplitude of approximately
on a millisecond time scale,5 after which most sodium channels are inactivated. During the upstroke, around
, voltage-gated calcium channels in the membrane slowly start to open. After a small but rapid repolarization caused by a transient outward K
current, the influx of Ca
into the cell, combined with decreased K
conductance and calcium-induced calcium release from internal storages such as the sarcoplasmatic reticulum, leads to the formation of a plateau phase of the membrane potential which lasts about
and is one of the main differences to other excitable cells such as neurons in the brain. Towards the end of the plateau, the potassium conductance increases again and the calcium channels close, which causes the cell to repolarize to its resting membrane potential. Only now, triggered by the value of the membrane potential, the sodium channels return from their inactivated to the (initial) closed state, which can be activated again to elicit another action potential. The initial persistence of the inactivated state is what causes the cell to be less excitable after an action potential has been initiated and is therefore mainly responsible for the refractoriness of the cell as an excitable system. In addition to the aforementioned ATPases, the Na
/Ca
exchanger in the membrane helps expelling excess calcium from the cell. Most of the calcium is, however, taken up by the sarcoplasmatic reticulum via the Ca
ATPase. For a detailed description of the contribution of individual ion channels and their gating mechanisms beyond the simplified picture given here, the reader be referred to an extensive review by Grant [23].












The macroscopic force of the cardiac muscle is caused on the microscopic scale by the contractile force of individual cardiomyocytes, which is produced by the interaction of myosin and actin filaments within the cells. The action of this contraction machinery is triggered by the high intracellular calcium concentrations arising during the plateau phase of the action potential (excitation-contraction coupling [24, 25]) and is delayed with respect to the electrical activity of the cell.
1.2.2 Cell-to-Cell Coupling
Activity initiated by the excitation conduction system introduced at the beginning of Sect. 1.2 can propagate from cell to cell in order to cause contraction of a macroscopic region of the muscle. This cell-to-cell coupling stems mainly from gap junctions—proteins providing a direct electrical connection between the intracellular spaces of two neighboring cells [26].6 In this way, one cell performing an action potential can provide the necessary superthreshold stimulation for its neighboring cells.7 From a viewpoint of a reduced modeling approach, this is a next-neighbor coupling scheme which leads, macroscopically, to a diffusive interaction and thus turns the cardiac muscle into an excitable medium. As cells surrounding an excited cell not only receive input from that cell but also act as a charge sink, the action potential shape during wave propagation is altered compared to that measured in isolated cardiomyocytes [29].
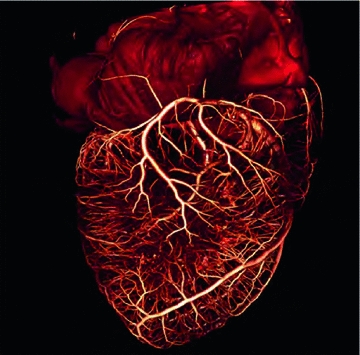
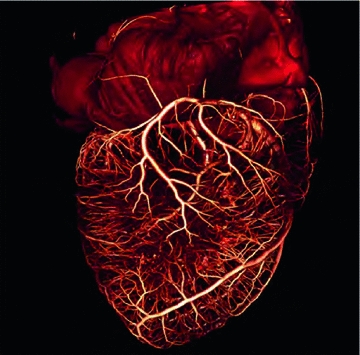
Fig. 1.5
The tree of cardiac vessels. Visualization of the branching network of coronary vessels of a whole beagle dog heart from a
CT scan after injection of contrast agent. The diameter of the heart is approximately
. Visualization and
CT data by M.L. Riccio/Cornell University
CT facility for imaging and preclinical research and F.H. Fenton/Cornell University




1.3 Structural Heterogeneity
The coupling between cells in cardiac tissue is inherently anisotropic, due to both the elongated, cylindrical shape of individual myocytes and the inhomogeneous distribution of gap junctions, which are found preferentially at the ends of the cell pointing in the longitudinal direction [26]. The anisotropic behavior of the tissue as a whole stems from the tendency of myocytes to locally align with their neighbors. However, this fiber orientation is not constant throughout the muscle, but rotates continuously from the endocardium to the epicardium by about
in total. Additionally, the cardiac muscle is organized in layers, so-called sheets, which are separated by regions of reduced coupling [30]. In the healthy heart, the anisotropy of the bulk electrical conductance manifests first of all in the anisotropy of conduction velocity [31]. Apart from the intracellular coupling, additionally, the ionic, i.e. local, properties of cardiac cells are known to differ across the ventricular wall and in different regions of the cardiac muscle, including dispersion in action potential duration and repolarization [32, 33].

The anatomy of the heart implies that a certain level of complexity and heterogeneity is always present: As described in Sect. 1.1, the coronary vasculature supplies the tissue with blood and removes waste products. The vessels form a tree-like network comprising the full range from very large vessels down to the smallest capillaries. An illustrative image obtained from micro-computed tomography (
CT) is shown in Fig. 1.5. Where these vessels penetrate the tissue, they disrupt the intracellular coupling between myocytes and therefore form non-conducting inclusions in the tissue, which can also be viewed as internal tissue boundaries. Other complex boundary shapes are introduced on the ventricular endocardium by surface undulations, papillary muscles and trabeculae (see Sect. 1.1 and Fig. 1.6).
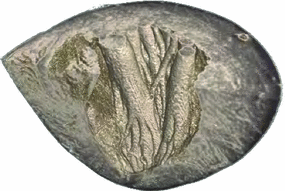

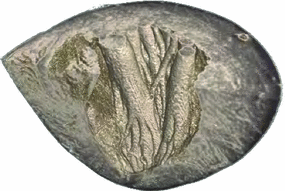
Fig. 1.6
Endocardial structure. Complex geometry of the inner ventricular wall of a mouse ventricle with a diameter of approximately
. Visualization and
CT data by D. Hornung


Besides this rather necessary and intended complexity of the cardiac muscle, further heterogeneity in the system is caused by random impurities such as fatty tissue, scars and fibrotic tissue, which may grow in number and intensity in the process of cardiac remodeling after myocardial infarction or due to other diseases. Fibroblasts occur naturally in healthy tissue as they are responsible for maintaining the extracellular matrix in which the cardiomyocytes are embedded. However, it has become clear that they not only influence the coupling between cardiomyocytes during development and tissue repair, but are also directly coupled to cardiac muscle cells and can therefore change the electrophysiological behavior of the tissue by acting as current sinks [34, 35].
1.4 Arrhythmias
Failure of the cardiac muscle to produce a normal heart beat or normal sinus rhythm can originate from different parts of the electrical conduction system of the heart. Besides the inability of the fast fibers to excite specific parts of the muscle (AV-block, bundle blocks, etc.), one important source of arrhythmias is the bulk of cardiac muscle tissue itself. Under normal conditions, the activation of a chamber of the heart can be caricatured as a coherent wave front, traveling across the tissue in a quasi-planar manner and thereby exciting the whole chamber. Afterwards, the muscle recovers and awaits the next triggered wave. However, waves that do not fully excite the available tissue volume at the first attempt can lead to the formation of reentrant wave patterns [36–38] (similar to those shown in Fig. 1.1). Once these self-excited spiral waves, or scroll waves in three dimensions, have been initiated, they excite the tissue with a frequency much larger than the natural pacemaker and thereby decouple a region from the control of sinoatrial node (via the mechanisms of high-frequency domination explained at the beginning of the introduction, see also Ref. [39]). If the ventricles are affected, spiral waves therefore lead to an unnaturally fast heart rate, called tachycardia in medical terms. Furthermore, the pumping function of the heart is impaired, since the contraction of the ventricles ceases to be coordinated with atrial activation and not the whole ventricle contracts at once. An even more dangerous situation arises when spiral waves break up into multiple waves, which may lead to a state of spatio-temporal chaos that is known as ventricular fibrillation [40, 41]. Under these conditions, small tissue regions contract in arbitrary sequence, causing the heart to completely lose its pumping force. The result is a standstill of circulation, called cardiac arrest, and consequently damage to the heart tissue itself, to other organs and, irreversibly within minutes, to the brain. Ventricular fibrillation thus is a lethal state of cardiac activity and its spontaneous occurrence is one of the main reasons for sudden cardiac death, which accounts for about 500,000 annual deaths in the United States [42]. Fibrillation can also occur in the atria, where it is not immediately life-threatening due to the filter effect of the atrioventricular node, which imposes an upper frequency limit on the excitation signal transmitted to the ventricles. However, even atrial fibrillation can cause serious long-term damage, e.g., by increasing the risk of stroke to five times its normal value [43].
The initiation mechanisms for arrhythmias are an active area of research. Most of the proposed mechanisms have in common that due to specific conditions, waves are partially blocked and some tissue region remains excitable for the potential formation of a reentry. One way this is possible is through premature beats (extrasystoles), where the partial block is caused by dispersion in refractoriness due to the preceding normal activation, acting as a dynamical heterogeneity. Alternatively, the substrate may have spatially inhomogeneous excitability, and this structural heterogeneity (see Sect. 1.3) can cause a partial block under stress conditions. For both possibilities, a number of underlying reasons have been identified, including, but not limited to, genetic mutations altering the dynamics of ion channels or receptors [44–46], remodeling after myocardial infarction [47, 48], electrical shocks during the vulnerable phase of a normal heart beat [49–52] or fibrosis [53–55]. A case which is difficult to classify is idiopathic ventricular fibrillation, which is thought to be responsible for a considerable percentage of sudden cardiac deaths and occurs in seemingly healthy patients [56].
1.5 Antiarrhythmic Therapies
There are at least three ways of treating cardiac arrhythmias: pharmaceutical intervention, ablation and electric shocks. While the first two can be used to reduce the susceptibility of the cardiac muscle to undesirable activation patterns, the latter cannot prevent arrhythmias from occurring. However, electrical defibrillation is the only therapy available for acute lethal arrhythmias such as ventricular fibrillation. Antiarrhythmic drug therapy can help controlling non-life-threatening arrhythmias or reduce the risk of arrhythmia onset, in particular for patients after myocardial infarction or suffering from atrial fibrillation [57, 58]. In some cases, a specific region in the heart can be identified as the cause of recurring arrhythmias. For example, certain types of atrial fibrillation have been shown to be caused by focal activity originating at the junction of the pulmonary veins and the left atrium. In radio-frequency ablation, a high-intensity current is used to deliberately create lesions around the foci and thereby electrically decouple the responsible region from the rest of the tissue [59, 60]. The same strategy is also applied to the ventricles, where areas that are “critical to the arrhythmia” [61] are destroyed. Apart from strong side effects of antiarrhythmic drugs that can damage other organs and possible (undeliberate) lesions of the heart from the ablation procedure, both antiarrhythmic drug medication and ablation bear the risk of proarrhythmic effects [62–64]. This is probably due to the indication-based prescription of specific drugs and the phenomenological choice of ablation sites, which are only rarely supported by a theoretical understanding of the mechanisms. In cases where proarrhythmic effects cannot be ruled out or if there is an irreducible risk of recurring ventricular arrhythmias, it is therefore appropriate to provide a “safety net” with the implantation of a device that can automatically deliver appropriate electrical shocks to terminate an arrhythmia [65].
To terminate the most lethal form of arrhythmia, ventricular fibrillation, implantable cardioverter-defibrillators (ICDs) as well as external defibrillators deliver a short, high-energy electrical shock.8 This shock (
within
if applied externally) is thought to excite the whole tissue, thus depriving the chaotic reentrant waves of the excitable substrate needed for propagation. In this way, all waves are terminated indiscriminately and the heart is shocked into quiescence. Afterwards, the low-frequency impulses of the sinoatrial node (see Sect. 1.2) can excite the heart and restore normal sinus rhythm. There is contradictory evidence whether the shock energies used in today’s defibrillators can cause considerable injury of the cardiac muscle [67, 68] and possibly increase the risk of future arrhythmias by introducing additional structural heterogeneity. A second important problem is that ICD therapy is also associated with severe psychological implications such as anxiety and reduced quality of life [69], mainly due to defibrillating shocks delivered during consciousness. These can be triggered due to early or mis-detection and are a traumatic pain experience for patients with implanted devices. Therefore, there is a search for low-energy alternatives to conventional defibrillation.


1.6 Complexity in Structure and Dynamics
As outlined above, the heart is a complex system in terms of structure, owing to its anatomy and heterogeneous bulk properties, as well as in terms of the (malignant) dynamics it exhibits. Obviously, the structural complexity forms the substrate for the dynamics, which is why the two kinds of complexity can interact across the whole range from the triggering of reentrant wave patterns, to their stabilization or further degeneration into spatio-temporal chaos, to their termination via pharmaceutical or electrical intervention. The dynamical complexity is thus only partly a result of the behavior as a generic excitable medium: planar waves representing normal activation, spiral waves associated with tachycardia and spatio-temporal chaos underlying cardiac fibrillation can be observed in simple, generic (and homogeneous) models of excitable media [70, 71]. The spontaneous occurrence of wave breaks necessary to produce arrhythmias in the first place, though, cannot be explained by such simplistic models. In contrast, the interaction of the dynamics with structural heterogeneities and the stochastic behavior of single cells have been shown to facilitate the occurrence of wave breaks and the development of reentrant waves [72–75]. These spiral or scroll waves waves can then interact with heterogneities, e.g. by pinning to inexcitable regions in the tissue [37, 76–78], by drifting due to excitability gradients [76, 79–81] or by destabilization due to the inherent and spatially varying anisotropy of cardiac tissue [82–84].
Over the last 20 years, it has become clear that the importance of the non-uniformity of the cardiac substrate extends to the mechanisms of electrical defibrillation. The simple picture given in Sect. 1.5 suggests that, in some way, most of the tissue is excited by a sufficiently strong electrical shock, which ultimately terminates all waves propagating through the muscle. In early efforts to understand the mechanism underlying electric-field induced activation of the tissue, this was thought to be possible because of the intracellular coupling scheme of cardiac tissue alone, where the voltage drop at high-resistance gap junctions leads to sawtooth-shaped depolarization pattern along a chain of interconnected cells. The spikes located at gap junctions were thought to be sufficient to initiate an action potential at one end of each cell [85–88]. However, several studies revealed that the expected depolarization patterns are not observed in real, well-connected tissue [89] and instead, additional heterogeneities in electrical conductance (including those on larger length scales) play a major role in the formation of these so-called secondary sources or virtual electrodes [90–96]. Electrical shocks above a critical defibrillation threshold of about
are believed to, on the one hand, activate a large enough tissue fraction by heterogeneity-induced virtual electrodes to terminate all existing waves and, on the other hand, minimize the risk of inducing new arrhythmias by interaction with preexisting activity [97, 98]. However, as most of the studies are focused on elucidating the mechanisms of conventional, high-energy, defibrillation, they are lacking perspectives for the development of gentler defibrillation techniques.

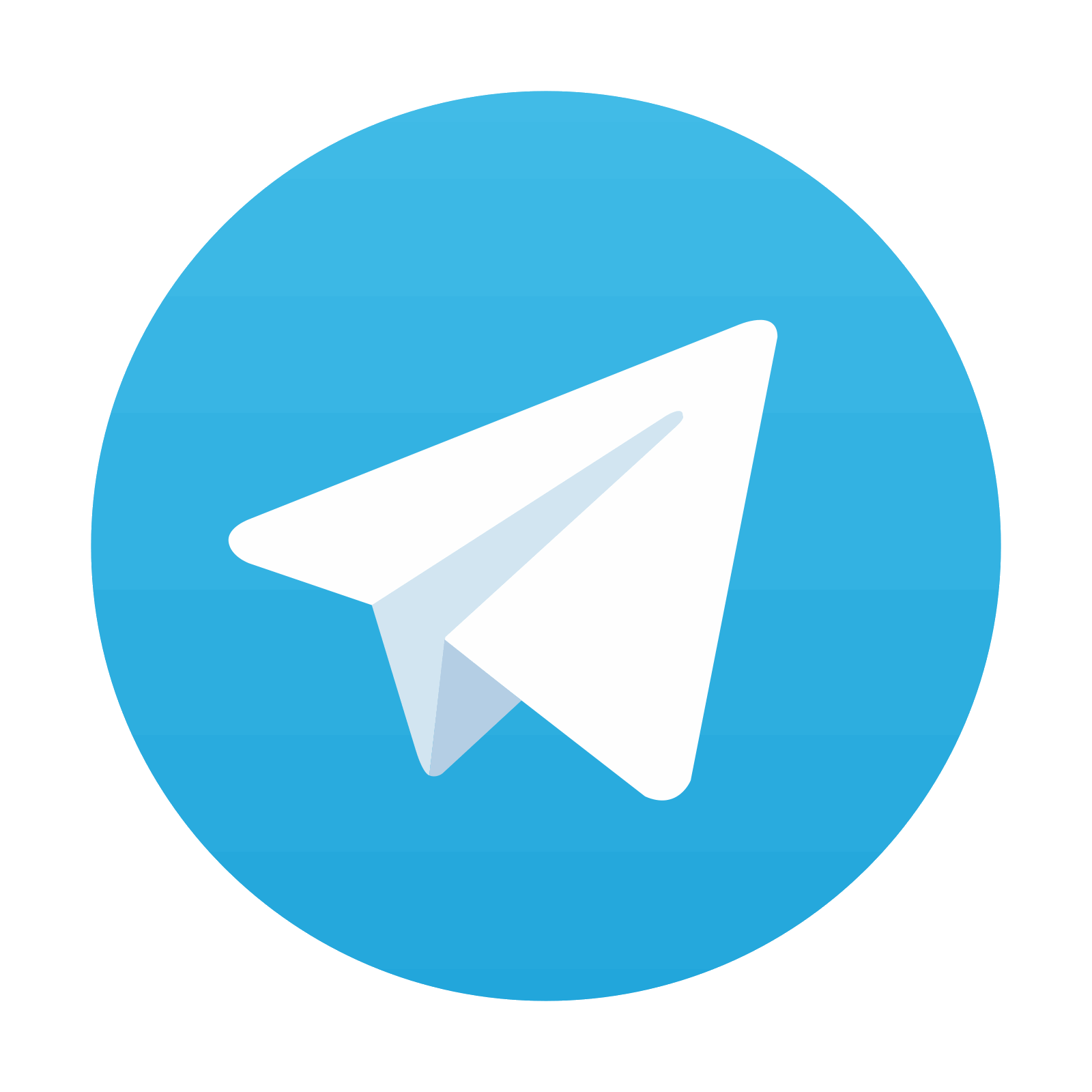
Stay updated, free articles. Join our Telegram channel
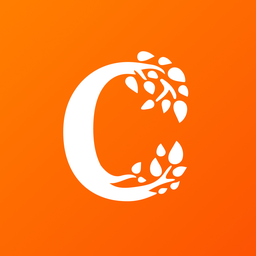
Full access? Get Clinical Tree
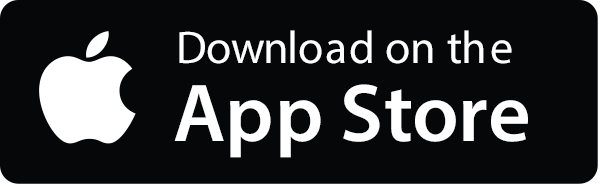
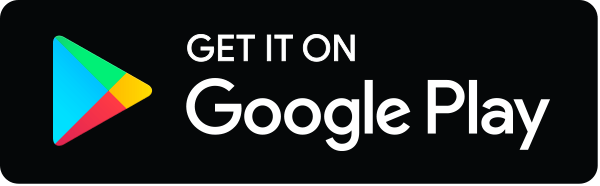