Interventional therapies for the treatment of heart rhythm disorders have rapidly evolved over the past three decades, and continue to go through significant evolution. Though management options for cardiac arrhythmias were previously limited to pharmacologic therapy, the transformation and adaptation of surgical procedures to less invasive catheter-based approaches have led to a new paradigm in arrhythmia management. A fundamental understanding of diagnostic and therapeutic strategies for treating heart rhythm disorders is critical to surgical specialties exposed to these rhythm disorders.
The recording of intracardiac signals through electrodes, and subsequent stimulation of the cardiac tissue, allowed for the concept of ablation. In 1967, Durrer and associates described reproducible initiation and termination of tachycardia in a patient with atrioventricular re-entrant tachycardia (AVRT) using a bypass tract.1 In 1969, the His bundle was first reproducibly recorded using a transvenous electrode catheter.2 The continued advancements allowing localization of intracardiac signals led to the study of a variety of tachyarrhythmias.
The concept emerged that critical regions of cardiac tissue were necessary for the initiation and propagation of tachyarrhythmias, and that if these regions could be interrupted, the tachyarrhythmia could be cured. Once catheter-based mapping strategies were developed to localize arrhythmogenic foci, surgical excision was contemplated. In 1968, a description of such a surgical procedure for the elimination of an accessory pathway (AP) was first published.3 This heralded an era of nonpharmacologic treatment of tachyarrhythmias.
A variety of arrhythmogenic foci and circuits were successfully mapped and ablated using surgical techniques in the 1970s. Resection of an atrial focus felt to be responsible for an atrial tachycardia was reported in 1973.4 Identification of reentry circuits within the atrioventricular (AV) node allowed surgical dissection to treat AV nodal re-entrant tachycardia (AVNRT) without causing complete heart block.5 Although surgical ablation was therapeutic for a variety of tachyarrhythmias, the morbidity and mortality associated with thoracotomy and open-heart surgery limited its widespread application. Because most supraventricular tachycardias (SVTs) are not life-threatening, the risk of the procedure limited its routine adoption. Surgical ablation was therefore limited to highly symptomatic patients refractory to medical therapy.
In order to minimize the morbidity associated with surgical ablation, a method of using a transvenous catheter for the delivery of energy directly to cardiac tissue was sought. In 1981, Scheinman and colleagues reported the first catheter-based ablation procedure, describing the ablation of the His bundle in dogs.6 This same group performed the first closed-chest ablation procedure in a human; in a patient with atrial fibrillation (AF) and rate control refractory to medical therapy, a transvenous catheter was advanced to the His bundle region. Using a standard external direct-current (DC) defibrillator, they attached one of the defibrillator pads to the intracardiac catheter and used the second defibrillator pad as a cutaneous grounding pad. A series of DC shocks was delivered between the two pads and complete heart block, and thereby rate control, was achieved.7
This closed-chest catheter-based procedure was quickly adopted to treat a variety of SVTs dependent on the AV node.8 As experience was gained and dedicated ablation catheters were developed, energy could be more precisely directed to allow ablation of APs, atrial tachycardia, single limb of AVNRT, and ventricular tachycardia (VT).
Although DC shock ablation allowed for the initiation of catheter-based ablation, it had various limitations. Because energy delivery was not titratable, such treatment had variable outcomes and complications, including massive damage to surrounding myocardium.6,7,9 Because DC energy was delivered from the intracardiac electrode toward a cutaneous site, general anesthesia was required.
The introduction of radiofrequency (RF) energy as an ablative energy source heralded a new era in the catheter-based treatment of arrhythmias. RF energy had been used for decades by surgeons for surgical cutting and cautery and had a long history of safety and efficacy. Animal studies using RF energy for the treatment of arrhythmia were first described in 1987.10 Intracardiac RF energy produces controlled lesions at the catheter tip using resistive heating.11,12 RF now remains the primary energy source for catheter-based ablation, though alternative energy sources such as microwave, laser, high-intensity focused ultrasound, and cryothermal energy continue to be developed as a means to optimize patient safety and clinical outcomes.13
Ablation using RF as an energy source involves the delivery of sinusoidal alternating current between the catheter tip at the endocardial surface and a large grounding pad on the skin. The current has a frequency of 350 to 700 kHz. The principal method of tissue injury with RF delivery is thermal. As the RF energy passes through the tissue at the distal electrode of the ablation catheter, resistive heating occurs at the catheter tip, and deeper tissue heating occurs through passive heat conduction, producing coagulation necrosis, and a discrete homogenous lesion. To achieve irreversible tissue injury, a tissue temperature of 50 to 58°C is required. Lesion size is proportional to the tissue temperature, size of the ablation electrode, contact force between the catheter and tissue, and duration of RF delivery.14 If subendocardial tissue temperature exceeds 100°C, steam may form within the tissue, resulting in a rapid expansion and crater formation and an audible “pop” during ablation. Much like DC shocks, such “steam pops” can cause unpredictable injury (eg, to surrounding normal conduction tissue) as well as local tissue rupture. Contemporary RF ablation catheters have a thermistor or thermocouple that allows for automatic adjustment of the power output to achieve a preset temperature at the electrode tip-tissue interface.
With endocardial ablation, the lesions produced are limited such that some intramural and epicardial foci and arrhythmia circuits may not be reachable with standard ablation. One method to increase lesion size and depth uses the principle of limiting coagulum development by increasing the electrode-tissue interface by increasing tip size.15,16 A limitation of larger catheter tips is that the larger surface area makes it difficult to regulate power delivery and achieve even temperatures.
Cooling the ablation catheter tip with saline irrigation, either within the catheter or external to the catheter, can also prevent coagulum formation at the tissue interface. This prevents a rise in impedance and allows for more energy delivery deep into the tissue.17,18 Irrigated RF catheters bathe the catheter tip internally using recirculating saline or externally through a porous electrode tip. These catheters continue to use RF as an energy source, with maximization of power delivery resulting in deeper lesions with a greater volume than with standard RF.19 Cooled epicardial RF ablation probes have been US Food and Drug Administration (FDA) approved, and transfers established catheter-based technology to a surgical approach for epicardial ablation of cardiac arrhythmias, used primarily in conjunction with other open cardiac surgical procedures.20,21
Because of concern about the irreversible nature of RF delivery, alternative energy sources have been developed that allow for a transient tissue injury before placement of permanent lesions. Cryoablation uses cryothermal energy delivered via a dedicated cryoablation catheter to produce gradual cooling of cardiac tissue to create local tissue damage. Hypothermia has been the preferred method of delivering linear lesions in surgical ablation. This technique uses pressurized nitrogen or nitrogen oxide flow through a catheter tip nozzle. As the gas expands beyond the obstruction, there is a temperature drop to as much as –90°C. The major advantage of cryothermal energy delivery relative to RF is its ability to deliver both transient and permanent injury to the tissue; the initial cooling phase (“Cryomapping”) allows one to assess not only the impact of cryoablation on pathologic tissue (eg, anteroseptal APs, the slow limb of a dual AV node), but also the impact of potential lesion placement on nearby structures such as surrounding normal conduction tissue.22 If the Cryomapping phase yields desirable results, then the temperature is lowered even further to a “freezing” stage. The ability to deliver reversible injury and catheter stabilization has made cryoablation increasingly popular in those cases in which the pathologic lesion is in close proximity to the AV node, and in younger patients in whom avoiding the need for pacemaker implantation is the highest priority.23
A second potential disadvantage to RF as an energy source is the risk of extracardiac tissue injury. For example, ablation of AF is becoming the most commonly performed electrophysiologic procedure, and is based on elimination or isolation of triggers for AF, which occur most commonly within the pulmonary veins (PVs). During catheter-based pulmonary vein isolation (PVI), ablation within the left atrium carries risks of collateral damage to surrounding structures, resulting in potentially highly morbid complications such as PV stenosis, atrioesophageal fistulas, and phrenic nerve paralysis.24,25 As the esophagus runs adjacent to the posterior wall of the left atrium, this structure is highly vulnerable during ablation of AF. Though the rate of clinical atrioesophageal fistulas after ablation of AF is very low (around 0.04%),26 the rate of intraoperative atrioesophageal perforation was as high as 1.3% in one open-chest series of patients undergoing linear lesions between PV ostia, to include the posterior wall overlaying the esophagus.27 Current ablation strategies include methods to monitor for esophageal heating, such as continuous monitoring of esophageal temperature with an adjustable esophageal temperature probe.
Diagnostic localization of tachyarrhythmias involves positioning catheters in strategic locations within the heart to obtain intracardiac recordings from various cardiac chambers as well as from the His bundle. Venous access is typically obtained in the bilateral femoral veins using the Seldinger technique. Catheters of 4 to 6 French in size are passed under fluoroscopic guidance into the right atrium and right ventricle, as well as positioned just across the tricuspid valve (TV) on the septum to obtain His bundle recordings. To obtain recordings of the left atrium and left ventricle (LV), a catheter is guided into the coronary sinus (CS), which passes posteriorly behind the mitral annulus and drains into the right atrium (Fig. 53-1).
FIGURE 53-1
Radiograph in the right anterior oblique projection showing catheters positioned for a standard diagnostic electrophysiology procedure. Three nonsteerable diagnostic catheters are introduced from the inferior vena cava into the right heart. Two 4-French catheters with four electrodes are positioned in the region of the right atrial appendage (RA) and right ventricular apex (RV apex). A 5-French catheter with six electrodes is positioned across the tricuspid annulus to obtain a His bundle recording (His). A nonsteerable 6-French catheter is introduced via the right internal jugular vein into the coronary sinus (CS) to obtain left atrial and ventricular recordings. Finally, a deflectable 7-French ablation catheter is positioned in the region of the low right atrium.

Direct recordings of the left heart are sometimes necessary and accomplished either by transseptal cannulation through the intra-atrial septum from the right atrium, or via a retrograde approach across the aortic valve into the LV, using catheters percutaneously placed into the femoral artery. Catheter and sheath placement within the left heart, however, imposes a risk of thrombus formation on the intracardiac catheter and at the site of ablation, with the resultant risk of stroke and thromboembolism. Animal models have determined that mural thrombus is evident in up to 50% of cases immediately after RF ablation.10,28 In addition to the risk of mural thrombus at the site of ablation, there are mounting evidence of a systemic prothrombotic condition after RF ablation.29,30 Therefore, during left-sided ablation, anticoagulation with intravenous heparin is typically administered throughout the procedure, and substantially reduces the thromboembolic risk. During ablation of AF, with the majority of the procedure performed within the left atrium, heparin is administered to achieve and maintain a target activated clotting time of 300 to > 400 seconds throughout the procedure.31 Many patients undergoing ablation, particularly for AF, are on chronic systemic anticoagulation. Historically anticoagulation with warfarin was held prior to the procedure, and the patient was bridged with heparin in the periprocedural period. This strategy has now been recognized to be associated with a high incidence of bleeding complications, and now ablations are routinely performed in patients on uninterrupted therapeutic anticoagulation. In the event of procedure-related bleeding complications such as cardiac tamponade, anticoagulation can be reversed with protamine, fresh frozen plasma, and/or prothrombin complex concentrate (PCC). While most experience has been with continuous warfarin, evidence is emerging that ablations can also be safely performed in the setting of anticoagulation with the novel oral thrombin inhibitor or Factor Xa inhibitors, though clinical experience is limited and options for reversal of anticoagulation, when necessary, are still in development.31
Once diagnostic catheters are positioned in preparation of an electrophysiologic study, programmed electrical stimulation is performed to induce and study the tachyarrhythmia. Sometimes modulation of the autonomic nervous system is required to induce tachyarrhythmias with the infusion of atropine, isoproterenol, or epinephrine.32 Once an optimal site for ablation is determined, a steerable ablation catheter is positioned at the target site, and energy is delivered.
At the end of the procedure, all catheters and sheaths are removed and manual pressure is applied to the access sites to achieve hemostasis. If the patient was heparinized for the procedure, sheath removal is delayed until anticoagulation reverses, either when the activated clotting time is less than 200 to 250 seconds, or after administration of protamine. The patient is placed on bed rest for 4 or more hours. Routine follow-up studies are not warranted unless required to assess for a complication. As mentioned, because of the risk of thromboembolic events, patients are frequently sent home on aspirin, thienopyridines, low-molecular-weight heparin, warfarin, or a combination of risk-appropriate antithrombotic therapies depending on the type and extent of ablation performed.
In referring patients for catheter ablation, it is important to weigh the risks and benefits of the procedure for the individual patient. Most tachyarrhythmias, although causing a variety of symptoms, are generally hemodynamically well tolerated and are not life-threatening. Thus awareness of the potential complications of catheter ablation is necessary before referring a patient. Complications can be divided into those involving vascular access, catheter manipulation within the heart, and ablation.
Access-related complications include pain, adverse drug reaction from anesthesia and sedation, infection, thrombophlebitis, bleeding at the site of access, hematoma, arteriovenous fistula, and pseudoaneurysm formation. Arterial damage or dissection may also result. Systemic or pulmonary thromboembolism can occur, most seriously resulting in transient ischemic attack or stroke. It is felt that performance of complex ablations while on systemic anticoagulation and with externally irrigated tipped catheters may reduce the risk of mural thrombus and thereby periprocedural embolic events. However, there may be an increased risk of access complications in a patient with therapeutic anticoagulation.33,34
Complications associated with placement of intracardiac catheters can be more life threatening. These include perforation of a cardiac chamber or the CS, resulting in hemopericardium, cardiac tamponade, and potentially coronary artery injury. Programmed electrical stimulation can result in the induction of hemodynamically unstable tachyarrhythmias such as VT or fibrillation. Catheter manipulation can also result in usually transient but sometimes permanent damage to valvular apparatus or the conduction of the right or left bundle branches owing to mechanical trauma.
RF delivery to cardiac tissue is associated with risk of collateral damage to intracardiac and extracardiac structures. Inadvertent ablation of the normal conduction system may result in complete heart block requiring permanent pacing. Perforation of a cardiac chamber or vascular structure can also occur with catheter manipulation or RF delivery; small perforations can often be adequately controlled with percutaneous pericardiocentesis and reversal of anticoagulation if present, however, if cardiac bleeding does not stop with these conservative measures an urgent open surgical approach is needed. Collateral damage to coronary circulation can result in myocardial infarction, heart failure, or cardiogenic shock. Phrenic nerve paralysis can occur due to ablation in proximity to the right or left phrenic nerves, which course along the pericardium. Ablation near the PVs within the left atrium can result in venous stenosis and pulmonary hypertension. Ablation in the posterior wall of the left atrium, as is routinely performed during ablation of AF, can result in damage to the esophagus, including esophageal ulcer formation and the potentially fatal complication of atrial-esophageal fistula.35 Given these risks, a center undertaking these procedures must be prepared to urgently treat these potential complication. All operators performing electrophysiologic studies should be trained in pericardiocentesis, and certain procedures associated with higher risks of perforation should only be performed at centers with available cardiac surgeons in the event of severe complications.36
An 8-year prospective study published in 1996 of 3966 procedures found an overall complication rate of 3.1% for ablative and 1.1% for diagnostic procedures, with complications more likely to occur in elderly patients and those with systemic disease.37 A more recent series evaluating ablations performed in patients across a series of age ranges identified a rate of procedural complications of 1.3%, with no significant differences between patients under 70 years of age relative to septagenarians or octogenarians.38 Various studies have shown very low mortality rates directly attributable to the electrophysiology study.
A variety of techniques have been developed to elucidate the origin and mechanism of tachyarrhythmia propagation. Some of these techniques involve pacing in sinus rhythm, whereas others are performed during the tachyarrhythmia. Diagnostic studies typically involve programmed electrical stimulation with pacing at particular intervals to initiate a tachyarrhythmia, assess its response to pacing maneuvers, or terminate it.39
Activation mapping is used to localize the origin of various tachycardias. It involves positioning a mapping catheter in various intracardiac locations during the tachyarrhythmia, to identify a site where activation is earliest, and precedes any other intracardiac activation or corresponding surface P wave or QRS. The earliest site of activation during a focal tachycardia must by definition be the source of the tachycardia.40,41 This technique is most useful for localizing focal atrial tachycardias, stable VT, and APs.
Entrainment mapping is used for localization of re-entrant circuits, and is often used in conjuction with activation mapping to identify targets for ablation. In entrainment mapping, pacing is performed from intracardiac sites during tachycardia and slightly faster, to penetrate the arrhythmia circuit and entrain the tachycardia when pacing from sites within the re-entrant circuit, the time from the last paced stimulus to the first return signal on the pacing electrode (called the “post-pacing interval”) should equal the tachycardia cycle length. Since you are pacing from within the circuit, one cycle results in the “return” signal which is the same of the tachycardia cycle length. Pacing from sites more remote from the circuit will produce a post-pacing interval greater than the tachycardia cycle length. Because pacing is not within the circuit, the time to the “return” signal is the sum of one revolution around the circuit (the tachycardia cycle length) plus the time it takes to get to the circuit and back from the circuit. The difference between the post-pacing interval and the tachycardia cycle length can be used to determine whether sites are within the arrhythmia circuit and therefore guide ablation. This strategy is particularly useful for mapping atrial flutter, re-entrant atrial tachycardia, and scar-related VT.42
Pace mapping is another method used to localize the origin of a tachycardia, with particular usefulness in mapping VT or ventricular ectopy.43 In this method, pacing is performed during sinus rhythm at various intracardiac locations, and the paced QRS morphology is compared to the QRS morphology during tachycardia; the disparity in QRS morphologies can be assessed and the catheter repositioned until a match is obtained. At the site of tachycardia origin or myocardial exit, pacing is likely to produce a QRS morphology identical to that observed during tachycardia since the remainder of the heart is being activated in a similar manner.44
Mapping may also be performed based on anatomical landmarks. For example, ablation of the slow AV nodal pathway for the treatment of AVNRT is performed by ablating well-defined targets identified by fluoroscopy with or without the assistance of an electroanatomic mapping system. In common right atrial flutter, in which conduction across the cavotricuspid isthmus (CTI) is necessary to sustain the arrhythmia, anatomical landmarks are used for ablation to create a line of block between the tricuspid annulus and the inferior vena cava (IVC).45
In various clinical situations, a combination of these mapping techniques may be used to localize an appropriate target for ablation.
The success of ablation is dependent on precise localization of arrhythmogenic foci and critical parts of arrhythmogenic circuits. Advanced mapping techniques have been developed as adjuncts to conventional mapping methods to improve the efficacy and safety of catheter ablation. The systems described below are useful to improve the rapidity and precision of mapping arrhythmias, while decreasing the need for fluoroscopy.
The CARTO electroanatomical mapping system (Biosense-Webster, Diamond Bar, CA) uses a magnetic field and emitted currents to localize a mapping catheter in three-dimensional space. Various locator pads placed beneath the patient’s chest generate ultra-low-intensity magnetic fields in the form of spheres that decay in strength. A sensor in the catheter tip measures the relative strength and hence the distance from each of the pads, triangulating the temporal-spatial location of the catheter. For activation mapping, electrodes at the catheter tip record local electrograms, and the local activation times acquired from various mapping points are reconstructed on a three-dimensional map relative to a reference catheter, and presented on the map in a color-coded fashion. By acquiring multiple mapping points during tachycardia, the sequence of arrhythmia propagation can be reconstructed. Voltage maps can also be obtained to delineate regions of scar and diseased myocardium.46
Another commonly used nonflouroscopic three-dimensional electroanatomic mapping system is the EnSite system (Ensite NavX and EnSite Velocity, St. Jude Medical, St. Paul, MN), which consists of surface electrode patches applied to several places on the patient’s body. To create a three-dimensional model of the chamber of interest in the heart, an electrical signal is transmitted between the patches, and the signal is sensed by the catheters within the heart; as the catheter is moved within the chamber, information from the intracardiac catheters including location, activation timing, and local electrogram amplitude is relayed to the computer system that generates the three-dimensional model. Cardiac electrical activity, including activation and voltage maps, can be superimposed on the anatomic model. The EnSite Velocity System can display the real-time position of conventional electrophysiology catheters, and can provide visualization and navigation of up to 128 electrodes on a combination of catheters.47
Intracardiac ultrasound catheters have had substantial benefits in improving the safety and efficacy of ablation, by providing real-time visualization of intracardiac structures. Radial array intravascular ultrasound (IVUS) (9 MHz Ultra ICE, Boston-Scientific, Natick, MA) may be positioned within the atria, allowing visualization of the interatrial septum, thereby enhancing the safety of transseptal punctures. Phased array intracardiac echocardiography (ICE) has extended the principles of IVUS for electrophysiologic use.48 Newer ICE catheters are steerable and have Doppler capability (Acuson, AcuNav, Mountain View, CA). In addition to guiding transseptal punctures, ICE catheters allow the accurate targeting of anatomical sites such as PV ostia and papillary muscles.49 They are also useful for imaging diagnostic and ablation catheter positions and visualization of tissue contact for optimal ablation. Additional benefit to ICE is integration with electroanatomical mapping systems to allow for noncontact real-time reconstruction of cardiac structures (CartoSound, Biosense-Webster, Diamond Bar, CA) (Fig. 53-2).
FIGURE 53-2
Integration of live intracardiac echocardiography (A) into the creation of a three-dimensional electroanatomic map of the left ventricle using the CARTO mapping system (CartoSound, Biosense-Webster, Diamond Bar, CA). (B) A voltage map of the left ventricle is shown in the RAO view, superimposed over the corresponding echocardiographic image.

Using the techniques described in the preceding sections, most focal and re-entrant tachyarrhythmias can be mapped and targeted for catheter-based ablation.
AVNRT is the most common form of SVT identified during electrophysiologic studies. This tachycardia can present at any age, although most patients who present for medical attention are in their forties and the majority are females.50,51 Advances in catheter ablation of this tachycardia has made it a first-line therapy for those symptomatic patients not wishing to take long-term medications.52
AVNRT has a re-entrant mechanism using two distinct electrical pathways within the AV nodal tissue. The common pathways are known as “slow” and “fast” based on their relative conduction properties. The anatomical location of these pathways is variable but generally located within the triangle of Koch in the septal right atrium, which is bounded by the tricuspid annulus and the tendon of Todaro with the CS at the base. The apex of the triangle is the His bundle at the membranous septum where it passes through the central fibrous body. The anterior third of the triangle contains the compact AV node and the fast pathway, and the inferior and posterior portion, near the CS ostium, is the typical location for the slow pathway (Fig. 53-3).53
FIGURE 53-3
(A) Diagrammatic representation of typical atrioventricular nodal re-entrant tachycardia. Surface ECG shows narrow complex tachycardia with no clear P waves. The re-entrant circuit (blue arrows) consists of the posterior slow pathway region acting as the antegrade limb, and the anterior fast pathway region acting as the retrograde limb. The slow pathway target site is located between the coronary sinus os (CS) and the tricuspid valve annulus (TV). IVC = inferior vena cava; RA = right atrium; RV = right ventricle; SVC = superior vena cava. (B) Surface ECG showing precordial leads in AVNRT. This demonstrates that the retrograde P waves are barely discernible in some leads. In V1, it forms a pseudo r′ wave (arrow). P waves are also visible in the terminal portions of QRS complexes in V2 and V3 but not in the lateral leads.

In the typical form of AVNRT, antegrade conduction from the atrium to the ventricle occurs over the slow AV nodal pathway, and the retrograde conduction through the AV node occurs over the fast pathway. Because conduction in the retrograde direction is fast, the atria and ventricle are depolarized almost simultaneously. Thus the electrocardiographic feature of this tachycardia is P waves that are inscribed within the QRS and thus not seen or barely discernible at the termination of the QRS complex.54 The arrhythmia is usually triggered by an atrial premature complex (APC). The refractory period of the fast pathway is longer than that of the slow. Under the right conditions, the premature impulse blocks in the fast pathway and proceeds antegrade down the slow pathway, resulting in marked PR prolongation (a “jump”). Since nothing came down the fast pathway the impulse can turn around and conduct retrograde up the fast pathway resulting in an atrial echo beat. If the slow pathway has recovered the tachycardia can continue.55 In fewer than 10% of cases, the circuit is reversed. In atypical AVNRT, antegrade conduction occurs over the fast pathway and retrograde conduction occurs over the slow pathway. Thus the ECG of this tachycardia shows inverted P waves in the inferior leads denoting retrograde activation of the atria with a longer RP segment owing to slower retrograde conduction.56 Less commonly other AV nodal circuits may be present such as “slow-slow” AVNRT, using antegrade conduction down one slow pathway, and retrograde conduction utilizing another “slow pathway,” with different functional and anatomic properties. These alternate AV nodal pathways occasionally have leftward extensions creating eccentric retrograde atrial activation patterns.55
Slow pathway ablation has a high degree of success for elimination of AVNRT, with a recurrence rate in the range of 2 to 7%. Complete AV block can occur as a complication of attempted slow pathway ablation in less than 1% (range 0 to 3%) of cases.57 The North American Society of Pacing and Electrophysiology (NASPE) self-reported surveys on 4249 patients who underwent slow pathway ablations had success rates of greater than 96% and complication rates of less than 1%.58,59
About 30% of SVTs are caused by AVRT. This is a re-entrant tachycardia using the AV node and an AP. These APs are muscular bundles or remnants of conductive tissue from embryonic development that span the normally electrically inert tricuspid and mitral valve annulus and provide an independent path of conduction between the atria and the ventricles outside of the AV node. AVRT is part of the Wolff-Parkinson-White (WPW) syndrome of ventricular pre-excitation and symptomatic arrhythmias. The most common APs connect the atrium to the ventricular myocardium, but other APs may connect the atria or AV node to the His-Purkinje system. APs can conduct in the antegrade direction, retrograde direction, or both. In sinus rhythm, in patients with antegrade conduction over the AP there is preexcitation of the ventricles. Ventricular activation is a result of “fusion” between activation over the AP and over the AV node. The degree of preexcitation is determined by the conduction velocity through the AV node and the time the sinus impulse takes to get to the AP. Preexcitation is manifested on the surface electrocardiogram by a short PR segment and slurring of the onset of the QRS, known as the delta wave. This slurring is due to slower “muscle to muscle” conduction in the ventricle from site of AP insertion. Absence of these findings does not exclude an AP. This may be due to a “latent AP” where there is antegrade conduction but the majority of the ventricle is being activated by the normal conduction system. This is most often seen in young patients with rapid AV nodal conduction and a far left lateral AP. In addition, up to 30% of AP conduct in the retrograde direction only (“concealed pathways”).60
The term “WPW syndrome” is the combination of ventricular preexcitation on the surface ECG and symptomatic palpitations. However, it is often used to refer to any patient with an AP and SVT. Patients with WPW typically present with palpitation caused by rapid heart rate. This may be the result of AVRT or any supraventricular arrhythmia, including AF, with resulting rapid AV conduction via the AP. Associated symptoms may be mild such as palpitation and shortness of breath, or as severe as syncope and sudden death.61,62 Sudden death is a very rare complication of rapidly conducting APs, and may be caused by ventricular fibrillation resulting from the extremely rapid ventricular activation over the AP during AF.
Indications for ablation of APs include patients with symptomatic AVRT or those with atrial tachyarrhythmias with rapid ventricular conduction who fail or do not wish to undergo medical therapy.62 Relative indications for ablations include asymptomatic patients with “high risk” APs that are capable of rapid conduction thus posing a small risk of sudden death as the initial presentation of AF. Similarly, patients in high-risk professions, those with family history of sudden death, or those mentally distraught over their condition may be candidates for ablation.63
In the typical or orthodromic form of AVRT, antegrade conduction from the atrium to the ventricle occurs over the AV node and retrograde conduction occurs over the AP. In this form of AVRT, the P wave in the tachycardia follows the preceding QRS complex, resulting in a “short RP” tachycardia but often a longer RP segment on the surface ECG than seen in AVNRT (Fig. 53-4). In the rare antidromic form of AVRT, antegrade conduction occurs over the AP with retrograde conduction over the AV node, or more commonly a second AP. This results in eccentric depolarization of the ventricle, producing a wide complex tachycardia with retrograde P waves that can be easily mistaken for VT with one-to-one ventriculoatrial conduction.
FIGURE 53-4
(A) Diagrammatic representation of atrioventricular re-entrant tachycardia. This macro re-entrant circuit (gray arrows) uses the AV node and an accessory pathway (AP), in this case a right lateral pathway. In orthodromic AVRT, antegrade conduction occurs over the AV node and retrograde conduction occurs over the AP. Because of the conduction delay from the His-Purkinje system through the ventricular myocardium to reach the AP, retrograde P waves are discernible after the QRS complexes (arrow). In antedromic AVRT, the re-entrant circuit is reversed and surface ECG shows P waves that closely precede the QRS complexes. CS = coronary sinus; IVC = inferior vena cava; RA = right atrium; RV = right ventricle; SVC = superior vena cava; TV = tricuspid valve. (B) Intracardiac recording of atrioventricular re-entrant tachycardia with termination of eccentric conduction over the accessory pathway during RF ablation. The tracing at 50-mm-per-second speed shows four surface leads (VI, II, I, and aVF) and intracardiac recording from catheters: ablation (ABL); His distal, mid, and proximal; as well as right ventricular apex (RVA). The first three beats of the tracing show evidence of eccentric conduction over an accessory pathway: short PR segment and delta wave. With onset of RF energy (RF On) from the ablation catheter positioned in the region of shortest AV conduction, conduction becomes normal within two beats, with normalization of the PR segment and loss of the delta wave.

An unusual form of AVRT utilizes a slowly conducting or decremental AP. Since conduction in both limbs, antegrade AV node and retrograde AP, is slow the tachycardia can be very stable and almost incessant. These tachycardias were misnamed the “permanent form of reciprocating junctional tachycardia” or PJRT. They tend to be relatively slow (100 to 140 BPM) and can be mistaken for sinus tachycardia. On the surface ECG this is a “long RP” tachycardia with a short PR interval and inverted P waves in the inferior leads. It is important to recognize this entity as the incessant elevated heart rate can lead to a tachycardia-related cardiomyopathy. These pathways are almost always posteroseptal in location and ablation results in normalization of ejection fraction in most cases.64
Additional unusual APs are those that conduct slowly in the antegrade direction, connect into the specialized conduction system, and support preexcited tachycardias with a wide QRS. These are known as Mahiem pathways in which there is a slowly conducting antegrade AP connecting from the atrium to a fascicle (atriofascicular pathway) or from the AV node to a fascicle (nodofascicular pathway).
The 1998 NASPE prospective catheter ablation registry reported on 654 patients with a 94% success rate.59 Success rates are lower (in the range of 84-88%) for septal and right free wall pathways.65-67 Mortality rates are less than 1% and nonfatal complications occur in about 4%.59 The major challenges of AP ablation are the location of APs near the normal conduction system and those that are epicardial in location. Ablation of pathways that are anteroseptal and midseptal in location carry a higher risk of causing complete heart block due to ablation near the compact AV node or His bundle. In these cases, alternate ablative energy sources such as cryoablation may offer a potentially safer alternative due to its ability to deliver reversible injury, permitting of the resulting effects, prior to irreversible lesion formation.22,68,69 However, the vast majority of these pathways can be safely ablated with standard RF energy.
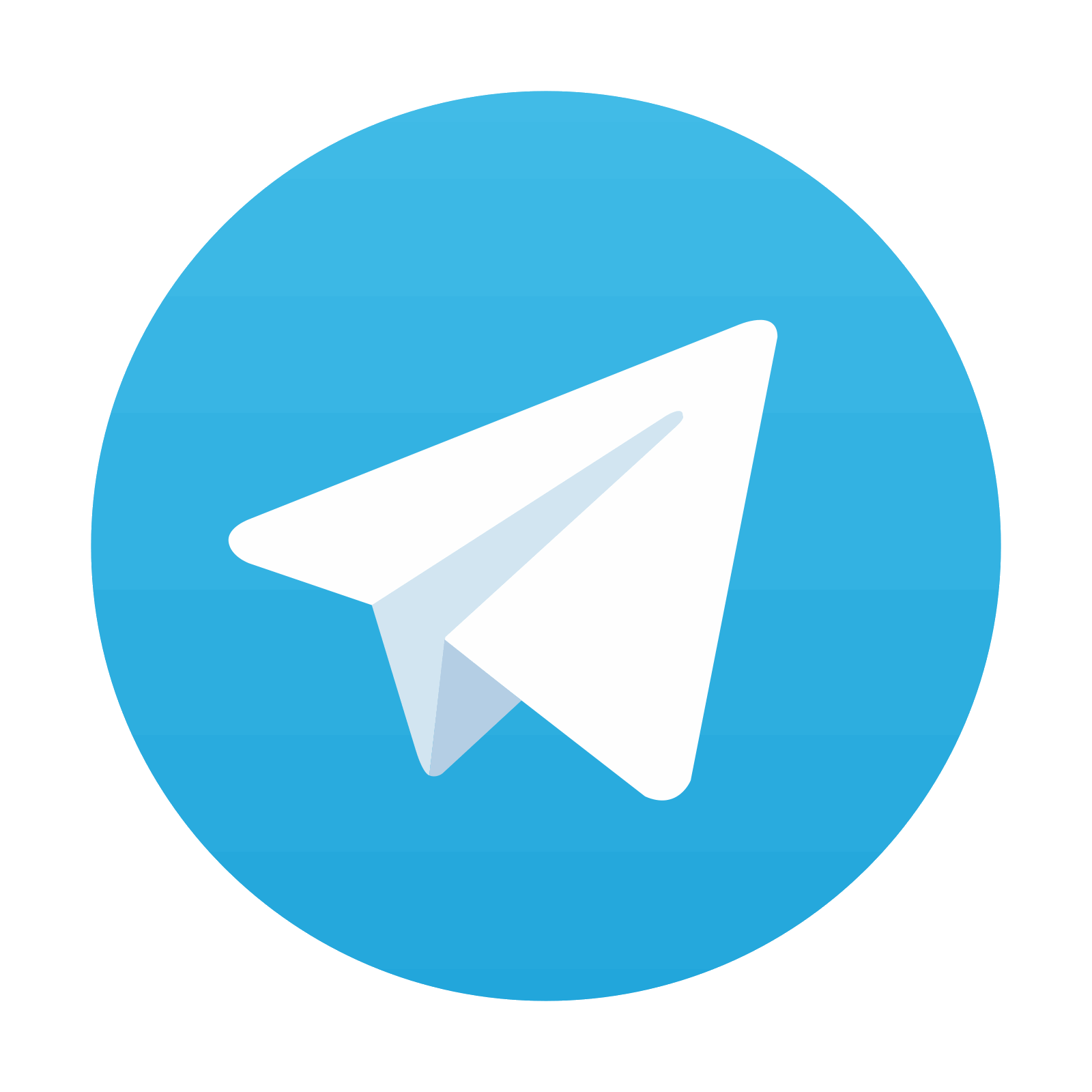
Stay updated, free articles. Join our Telegram channel
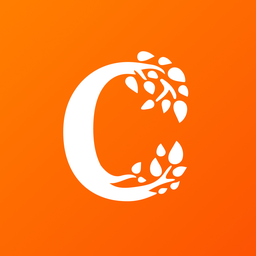
Full access? Get Clinical Tree
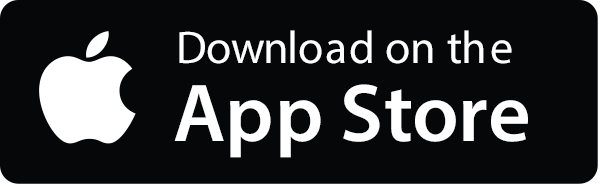
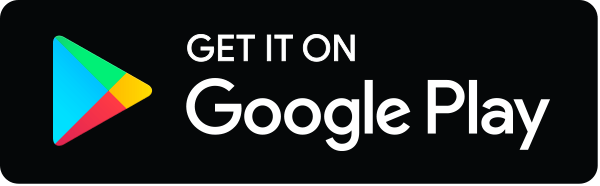