300 ms) can benefit from pacing.
Pacemakers also are used to treat advanced heart failure with major intraventricular conduction disorders, mainly left bundle-branch block (biventricular pacing for resynchronization).
Automatic calibration leads to adjustments of pacemaker response to normal changes in subject activity. Detection and dynamic analysis of nodal tissue rhythm provide accurate discrimination between simple acceleration and arrhythmias. An expert system associated with the pacemaker provides data for follow-up. Miniaturization of implantable pacemakers allows slightly invasive procedures. Safety features of pacemakers avoid the occurrence of induced currents during magnetic resonance imaging. Transient malfunctions of pacemakers during computed tomography can occur due to X-rays rather than small alternating electrical field (∼ 150 V/m) and alternating magnetic field (∼ 15 μT) [753].
Cardiac resynchronization therapy (CRT) is used in dyssynchronous heart failure (DHF). The CRT pacemaker possesses a third lead positioned in a vein on the outer surface of the left ventricle (RA, RV, and LV leads) [369]. Hence, both ventricles are electrically stimulated (paced) at the same time to support a synchronized contraction (biventricular pacing). Another type of implantable heart failure heart devices combines a CRT pacemaker with a defibrillator (CRTD).
The rhythm management relies on optimal left ventricle pacing lead placement. The risks and complications associated with the implantation of a CRT device are similar to those associated with the transvenous implantation of a conventional permanent pacemaker or implantable defibrillator [368]. These risks include bleeding, infection, pneumothorax, pericardial effusion, myocardial infarction, stroke, and death. Transvenous implantation of a left ventricular lead for CRT is accomplished via the coronary sinus and its tributaries. The specific risks comprise coronary sinus dissection and perforation, lead dislodgment, and extracardiac stimulation.
9.2.2 Electroporation-Based Treatment
Different methods have been proposed to stop the proliferation of vascular smooth myocytes, such as cryoplasty, or cryoballoon angioplasty,2 intravascular brachytherapy, or internal radiotherapy,3 vascular photodynamic therapy (VPDT),4 and drug-eluting stents.
Irreversible electroporation (IRE) is a nonthermal, nonpharmacological cell ablation method that uses a sequence of electrical pulses (e.g., sequence of 10 dc pulses; amplitude 3800 V/cm; pulse duration 100 μs; frequency 0.1 Hz) to produce permanent damage to tissue within a few seconds [754]. These pulses irreversibly create pores in the plasma membrane and cause cell death. An optimal value of IRE enables vascular smooth myocyte ablation, preserves arterial scaffold, and allows enabling endothelial regeneration. Endothelium integrity recovers.
9.3 Surgery
Surgery now uses robotic and imaging systems [755]. Virtual reality-assisted surgery planing (VRASP) has been developed to provide flexible computational support preoperatively [756] and peroperatively, which can control large patient-specific datasets in real time.
9.3.1 Cardiac Surgery
Cardiovascular surgery deals with congenital heart defect repair, valve replacements, heart transplantation, pacemaker placement, normal rhythm and/or conduction restoration (arrhythmia surgery, i.e., Maze procedure for atrial fibrillation), and coronary revascularization.
The ductus arteriosus is a vessel that connects the pulmonary artery and aorta in the fetus to shunt immature lungs, gas exchanges occurring in the placenta between fetal and maternal bloods. Shortly after birth, when the neonatal lungs carry out blood oxygenation, the ductus arteriosus closes. However, in about 1 in 2000 nonpremature infants, the ductus arteriosus remains open (patent ductus arteriosus), causing vascular diseases.
Many other types of heart malformations or congenital heart diseases exist, such as ventricular and atrial septal defects, atrioventricular canal, arterial stenosis, coarctation of the aorta, vessel transposition, cardiac valve atresia, hypoplastic left heart syndrome, tetralogy of Fallot, among others. Most defects are effectively treated by surgical and heart catheterization procedures.
Cardiac pacemakers are implanted into damaged hearts to correct defects in conduction of the action potential. Sleep apnea is current in adults with pacemakers. Nearly two thirds of patients with implanted pacemakers have obstructive sleep hypopneas and apneas. Patients with pacemakers thus should be routinely checked, because untreated sleep apnea contributes to cardiovascular deterioration [757].
Cardiac transplantation is the choice therapy for evolutive heart failure.Astrategy to treat severe heart failure targets cell growth and tissue regeneration or replacement, which are in a relatively early stage of development. As the donor pool for cardiac transplantation is insufficient, the alternative strategy relies on implantation of left ventricular assist devices (LVADs).
9.3.1.1 Ventricular Assist Pumps
Ventricular assist devices are mechanical circulatory support elements aimed at unloading the heart and providing adequate body perfusion, hence at improving the survival of patients suffering from heart failure and extending the time to transplantation.5
LVADs target patients with heart failure at a terminal stage waiting for transplantation, individuals with myocarditis and cardiomyopathy expecting complete cardiac recovery, and children in chronic stages of congenital heart disease who develop ventricular failure.
They include both portable extracorporeal and implantable pumps for patients with competent lung function. Extracorporeal membrane oxygenation is a short-term support when heart and lung failures are combined.
Device implantation can be associated with adjunctive therapy to improve ventricular recovery. Ventricular assist devices can be used not only as a bridge to transplant but also as a long-term (many years) aid.
A noticeable number of patients with LVADs later require right ventricular support. Patients with a LVAD must permanently take anticoagulants to prevent blood clot inside the pump. Complications occur, such a wire-mediated infection, device failure, hemolysis, infarction by cell fragments.
Second-generation LVADs are smaller than first-generation pulsatile machines. They rely on a rotor that continuously conveys blood without requiring valves. Thirdgeneration devices based on magnetically levitated rotors to reduce moving parts are under investigation.
A transcutaneous energy transfer system sends the energy across the skin via electromagnetic waves from an external coil to an electromagnetically coupled internal coil that charges an internal battery. This device thus avoids skin-piercing tubes.
The design of the device configuration and its wall surface must suit hemodynamic and hematological criteria [758]. LVADs were designed with an optimal size to be permanently implanted in the heart chamber, using minimally invasive procedures. Various cannula designs and diameters are proposed to match patient anatomy. LVADs must minimize hemolysis and thrombosis, as well as heat generation.
Artificial chambers are composed of two compartments, driving and blood chambers, separated by a flexible membrane [759]. The blood cavity has valved entry and exit orifices. Flow motion in the blood cavity is determined by membrane displacement controlled by the pressure in the second cavity. Numerical experiments have been performed in 2D models of these pumps [760, 761]. Once the existence and uniqueness of solutions of an elasticity problem in large displacement and small strain coupled with a viscous fluid are proved, numerical applications in an artificial heart ventricle used either a simplified arbitrary Eulerian–Lagrangian (ALE) method or the immersed boundary method. Diaphragm-type ventricular assist devices have also been studied experimentally (LDV) and numerically (using ADINA software) [762, 763].
Such a ventricular assist device is too big to be implanted in the circulatory system. Consequently, micropumps have been devised that can be implanted either in the left ventricle or between the left ventricle and the aorta. However, small pumps designed to be implanted in the left ventricle do not produce pulsatile flow (continuous-flow ventricular assist devices). Centrifugal pumps and pneumatic pulsatile ventricular assist devices have been proposed to be implanted in the body of both adults and children. Pump optimization and design methodologies have been based on 3D numerical simulations combined with design of experiments (DOE) [764], to develop a wholly implantable pump. Scaling has been set for an operating point using the Cordier diagram.
Implantation in any cardiac chamber or vascular lumen of mechanical devices, such as ventricular assist pumps, cardiac valves, and filters, among others, induces hemodynamic disturbances and damages flowing cells, causing in particular hemolysis. The implanted devices thus must minimize hemolysis.
Hemolysis indices have been proposed, such as a hemolysis index (HI), which measures the plasma-free hemoglobin (Hb) concentration
, a normalized hemolysis index (NHI) relative to total blood
, assuming that the hemolysis rate is small (
) and varies linearly with time (
) [765]:
![$$\mathrm{HI}\,\propto\,\frac{d[\mathrm{fHb}]}{dt}\,\frac{V_{\mathrm{pl}}}{q};$$](/wp-content/uploads/2016/07/A320740_1_En_9_Chapter_Equ1.gif)
![$$\mathrm{NHI}\,\propto\,\mathrm{HI}/[\mathrm{Hb}],$$](/wp-content/uploads/2016/07/A320740_1_En_9_Chapter_Equ2.gif)
where
is the plasma volume.
![$[\mathrm{fHb}]$](/wp-content/uploads/2016/07/A320740_1_En_9_Chapter_IEq3.gif)
![$[\mathrm{Hb}]$](/wp-content/uploads/2016/07/A320740_1_En_9_Chapter_IEq4.gif)
![$[\mathrm{fHb}]/[\mathrm{Hb}]\,\ll\,1$](/wp-content/uploads/2016/07/A320740_1_En_9_Chapter_IEq5.gif)
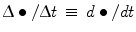
![$$\mathrm{HI}\,\propto\,\frac{d[\mathrm{fHb}]}{dt}\,\frac{V_{\mathrm{pl}}}{q};$$](/wp-content/uploads/2016/07/A320740_1_En_9_Chapter_Equ1.gif)
(9.1)
![$$\mathrm{NHI}\,\propto\,\mathrm{HI}/[\mathrm{Hb}],$$](/wp-content/uploads/2016/07/A320740_1_En_9_Chapter_Equ2.gif)
(9.2)

A prediction model was derived from the Giersiepen–Wurzinger blood damage relation using numerical simulations of blood flow field in the vasculature domain with the implanted device, introducing a pointwise function
, the damaged RBC fraction, which is supposed to depend on shear (
) magnitude and linearly on exposure time:6

where
.7



(9.3)

9.3.1.2 Valve Prostheses
“ Surgeons are not basically concerned with lesions. We care more about function. Therefore one may define the aim of a valve reconstruction as restoring normal valve fonction rather than normal valve anatomy.”
(A. Carpentier) [766]
Identification of mechanisms causing valve dysfunction is mandatory to successfully repair the valve. A complete valve analysis supports selection of appropriate repair techniques and obviates immediate and late repair failure.
Smooth, thin, and large leaflets are usually considered as repairable. Small, restrictive, fibrous, or thickened leaflets impede surgical repair. Heavily calcified valves are usually considered as nonrepairable, except when calcifications localize to the free margins.
Malfunctioning valves can be either repaired or removed and then replaced with prosthetic heart valves. Two main types of heart valve prostheses exist: mechanical valves and prosthetic stentless and stented tissue valves.
Mechanical valves are manufactured using three main designs:
1.
Caged ball valve (e.g., Starr–Edwards valve)
2.
Tilting disc valve (e.g., Medtronic Hall and Bjork–Shiley valves)
3.
Bileaflet valve (e.g., St. Jude, CarboMedics, and ATS valves)
Valved grafts have also been proposed (e.g., St. Jude aortic valved grafts and ATS aortic valve graft prostheses). Bioprosthetic (xenograft) valves are made from porcine valves (e.g., Carpentier–Edwards and Hancock valves) or bovine pericardium (e.g., Ionescu–Shiley and Carpentier–Edwards valves).
A collapsible trileaflet membrane valve is designed with two cylindrical muffs fixed together, a stiff and a thin flexible, the latter having collapsible cusps [767]. Its mechanical behavior must minimize regurgitation and pressure drop across it.
Prosthetic valves must mimic the static and dynamic characteristics of natural human valves and flow mechanics must be preserved, avoiding turbulences, flow stagnation, or excessive mechanical stresses.
Several teams have studied in pulsatile flow conditions the mechanical features of the valve prostheses using rigid [768, 769] or deformable [770, 771] test sections, which model either the local valvular region or the heart cavities, possibly with models of existing vessels [772].8 The gradual closure of the natural valves during flow deceleration is, in general, not reproduced.
Severe aortic stenosis are treated by catheter-based aortic valve insertion rather than surgical valve replacement. Less-invasive endovascular procedures, i.e., catheter-based implantation of aortic stent grafts and balloon- or self-expandable valve prostheses, use a retrograde femoral or apical route. Complications of catheterbased valve insertion comprise vascular injury, impaired device positioning with paravalvular leakage, cardiac tamponade, arrhythmias, coronary artery occlusion, and prosthetic valve embolization.
Anatomical and physiological explorations mainly based on echocardiography before conventional open-heart valve surgery aim at describing the leaflet anatomy and at assessing severity of valve dysfunction and hemodynamic consequences. The aortic root consists of the elliptical aortic annulus (transition between the left ventricular outflow tract and aortic root), commissures of aortic valve leaflets that extend upward, sinuses of Valsalva, coronary artery ostia, and sinotubular junction, which can also contain calcifications. The length of the aortic valve leaflet often exceeds the distance between the annulus and the ostium of the coronary artery, in the absence of strong aortic root remodeling associated with calcified aortic stenosis.
In the absence of direct operative inspection, three-dimensional, pre- and perinterventional imaging assists therapeutic planning with the detailed anatomy of the aortic valve and root as well as evaluation of vascular access strategy.
9.3.2 Coronary Arterial Surgery
In the presence of arterial stenoses, understanding of atherosclerosis evolution, diagnosis at relatively late stage of development, occurrence of complications in patients with moderate stenoses and not only severe ones, as well as the respective role of normal and tangential hemodynamic stresses are aspects that stimulate investigations by biomechanicians and biomathematicians involved in the implementation of atherosclerosis models as well as computer-aided medicine tools.
Late-stage intimal hyperplasia develops after grafting at the distal anastomosis and can cause restenosis, that is, renarrowing of the arterial lumen (Vol. 8, Chap. 8. Atherosclerosis—Medical Aspects). Many biomechanical studies were aimed at optimizing the neovasculature design to prevent this complication.
9.4 Endovascular Therapy
Endovascular techniques are aimed at treating local wall damage in the heart or blood vessels. Percutaneous interventions are minimally invasive because they use natural paths. The catheter is inserted into a superficial artery, such as the femoral or subclavian artery, and then advanced under image guidance into the diseased segment of the cardiovascular system.
9.4.1 Thermal Ablation of Nodal Tissue
Antiarrhythmic drugs are only partly effective in treating atrial fibrillation. Moreover, they have side effects such as arrhythmia. Paroxysmal atrial fibrillation triggered by focal drivers usually situated in a pulmonary vein can be cured by a catheter-based ablation.
Tachyarrhythmias can be treated by radiofrequency ablation (RFA). A selected small patch (
5 mm) of nodal cells of the conduction paths responsible for the abnormal cardiac rhythm is destroyed by a radiofrequency wave using an imageguided catheter-based procedure.

Cryoablation can also be used, providing cold to freeze and destroy cells.9 Damage limited to the local vasculature (without repercussion on the organ perfusion) can lead to ischemic necrosis, thus contributing to treatment efficacy. At a few millimeters from the ice ball edge, the tissue temperature is warmer, thus avoiding lethal tissue damage.
Ablation is proposed to treat reentrant tachycardias due to an extrapath in or adjacent to the atrioventricular node, junctional tachycardias, atrial flutter and fibrillation, ventricular fibrillation, and Wolff–Parkinson–White syndrome.
9.4.2 Drug-Eluting Stents
Although the likelihood of restenosis is reduced with respect to balloon angioplasty, stents are still prone to occurrence of intimal hyperplasia and restenosis. Drugs (e.g., rapamycin and paclitaxel) are stored in stent polymeric coating and, upon implantation, are locally slowly released, further reducing the restenosis rate.
Drug-eluting stents have a dual purpose: mechanical (like BMSs) and therapeutic to prevent restenosis by preventing smooth myocyte proliferation. The first generation of drug-eluting stents (DES) were aimed at addressing the high restenosis rate after implantation of bare metal stents. The goal of following DES generation with thinner struts and biocompatible or biodegradable polymers was to tackle the late stent thrombosis.
Antiproliferative drug-eluting stents (DES) include drug (cytostatic compound) reservoirs (coating thickness 5–20 μm). The main components that determine the performance of a drug-eluting stent are its structure and rheology as well as constituent drug, polymer, and delivery mode (Tables 9.1 and 9.2). Strut embedment in the arterial wall ranges from simple contact to total inclusion, thereby mildly affecting the drug delivery in the arterial wall.
Table 9.1
Strut and polymer thickness (μm) in different types of metallic stents. (Source: [773])
Type | Strut | Polymer | Total |
---|---|---|---|
Cypher select | 140 | 14 | 154 |
Taxus element | 132 | 16 | 148 |
Xience V | 81 | 8 | 89 |
Resolute | 91 | 6 | 97 |
Biomatrix | 120 | 11 | 131 |
Table 9.2
Some material constant of a stent and its coating. (Source: [774])
Elastic modulus (N/m ![]() | Poisson ratio | |
---|---|---|
Stent | 0.193 | 0.3 |
Coating | 0.01 | 0.3 |
To achieve a prescribed drug release kinetics over a given therapeutic period using a given dose, the current design strategies focus on a multiphasic release from blends of biodegradable polymers. A biphasic strategy, a first phase of immediate drug release liberate an adequate dose fraction and a second extended release provides the dose fraction required to maintain an effective sustained action, once the drug pharmacokinetics and pharmacodynamics are known.
The release pattern of paclitaxel from three neat polymer matrices, polycaprolactone (PCL), poly(lactic-co-glycolic) acid (PLGA), and PLGA polyethylene glycol (PLGAPEG) was studied [775]. These matrices are representative of a broad spectrum of biodegradable hydrophobic and hydrophilic polymers [776]. In hydrophilic polymers, the internal bounds between the chains are weakened, hence facilitating surface erosion.
Although DESs reduce the restenosis rate as they prevent vascular smooth myocyte proliferation, they provoke late-stage thrombosis resulting from endothelial injury associated with delayed or absent healing.
The same cause producing the same effect, these implanted materials damage the endothelium, especially at the insertion site, and can provoke in-stent restenosis despite braking smooth myocyte proliferation as they do not support endothelium repair. Endothelium damage and removal occurring during implantation induce a loss of a local, natural controller that impedes unwanted cell proliferation and provides an interface preventing unneeded blood coagulation. Antiproliferative drugs attenuates the division rate not only of smooth myocyte, but also that of endotheliocytes.
9.4.2.1 Thrombosis
Drug-eluting stents preclude not only in-stent restenosis, but also reendothelialization, hence predisposing to thrombosis. Poststenting vascular injury comprises endothelial denudation and stretching of the locally stiff vascular wall with its risk of tear. Endotheliocyte removal exposes the underlying procoagulant materials and eliminates the local endothelial control of vascular homeostasis.
Unlike stent thrombosis after implantation of a bare metal stent, which generally occurs within the first 2 weeks, late (after
\!\!1$
” src=”/wp-content/uploads/2016/07/A320740_1_En_9_Chapter_IEq21.gif”> year) stent thrombosis can appear after implantation of drug-eluting stents.
The main factors of stent thrombosis include [777]: (1) stent parameters (diameter, length, strut thickness, and polymer type and thickness); (2) patient status (ejection fraction, diabetes mellitus, age), because diabetes, renal insufficiency, and heart failure, predispose to stent thrombosis; (3) endothelium state.
Repair of the endothelial monolayer is required to struggle against thrombogenicity of the stented vessel segment. Damaged endotheliocytes are renewed by healthy adjacent cells and/or circulating endothelial progenitor cells originating from the bone marrow. Implantation of drug-eluting stents causes delayed arterial healing with poorer endothelialization and persistent fibrin deposition with respect to that of bare metal stents. Furthermore, patients who experienced stent thrombosis have higher platelet reactivity evaluated by ADP-induced platelet aggregation in comparison with a matched group of patients who underwent stenting without developing thrombosis.
Endothelial progenitor cells participate in reendothelialization. Late outgrowth endothelial progenitor cells, but not early outgrowth endothelial progenitor cells, differentiate into endotheliocytes. The number of circulating VEGFR2+ and PromL1+ (prominin-like-1 or CD133+) or CD34+ (sialomucin) cells that have the potential to differentiate into mature endotheliocytes is significantly reduced in patients suffering late (
-integrin [779].
![$>\!30$
” src=”/wp-content/uploads/2016/07/A320740_1_En_9_Chapter_IEq22.gif”></SPAN> d) stent thrombosis [778]. Moreover, endothelial progenitor cells are dysfunctional. Erythropoietin and vascular endothelial growth factor can enhance the number of circulating endothelial progenitor cells.</DIV><br />
<DIV class=Para>Recruitment of endothelial progenitor cells can be assisted by a GSK3β inhibitor, as their adhesion is enhanced via upregulated expression of the α<SPAN id=IEq23 class=InlineEquation><IMG alt=](/wp-content/uploads/2016/07/A320740_1_En_9_Chapter_IEq23.gif)
9.4.2.2 Restenosis
After stenting, medial smooth myocytes are much more easily exposed to circulating growth factors and inflammatory cytokines, such as platelet-derived growth factor, thrombin, thromboxane-A2, and adenosine diphosphate released in particular from platelets. Thrombin and PDGF stimulate vSMC proliferation and migration. Adenosine diphosphate binds to the platelet P2Y
receptor and potentiates platelet activation. Furthermore, dilation of the vascular wall activates stretch-activated signaling that provokes further vSMC migration and proliferation.

Among the mechanisms that elicit smooth myocyte proliferation and migration, calcium influx through Ca
ion channels such as store-operated channels is a major player. In addition to members of the family of transient receptor potential canonical (TRPC) channels, stromal interaction molecule STIM1 senses Ca
store depletion and works with Orai1 (Vol. 3, Chap. 3. Main Sets of Ion Channels and Pumps). Sensor STIM1 regulate directly TRPC1, TRPC4, and TRPC5 and indirectly TRPC3 and TRPC6. After arterial injury caused by angioplasty, STIM1 is overexpressed [780]. Conversely, suppression of STIM1 expression prevents intimal hyperplasia. Inhibition of STIM1 lowers the amount of phosphorylated retinoblastoma protein that regulates the G1–S transition of the cell cycle, thereby blocking cell cycle progression.


Several types of drug-eluting stents have been tested in clinical trials to prevent, or at least to limit SMC migration and proliferation into the intima, which lead to restenosis. Two main types of drug-eluting stents exist: (1) a more or less uniform coating by drug–polymer matrix, with a possible screen (topcoat) to reduce the transport speed and loss in blood flow; and (2) a set of drug-polymer reservoirs (size < 100 μm) in the metallic structure.
Rapamycin (sirolimus) and paclitaxel (taxol) are used as anticancer chemotherapy. Antiproliferative stent coatings employ these drugs (sirolimus- [SES] and paclitaxeleluting stents [PTES]). Rapamycin and paclitaxel target the G1 and M phases of the cell division cycle, respectively.
Rapamycin is an immunosuppressor used to prevent rejection in organ transplantation, as it supresses activation of B and T lymphocytes; it has potent antiproliferative properties, as it binds to FKBP12; the FKBP12-rapamycin complex then connects to and inhibits target of rapamycin, that is, the PI3K–TOR pathway.
Taxol is a microtubule-stabilizing agent with potent antiproliferative activity. Proliferation of vascular smooth myocytes can also be hindered by the inhibition of fibronectin matrix assembly [781].
Mitofusin-2, a member of transmembrane GTPases (MFn1–MFn2) of the outer mitochondrial membrane involved in mitochondrial fusion as well as maintenance and action of the mitochondrial network, has an antiproliferative effect, especially in vascular smooth myocytes, as it inhibits the Ras–ERK signaling and subsequently provokes cell cycle arrest at the G0–G1 transition [782].
Rapamycin also directly activates platelets, thereby contributing to thrombus formation. Therefore, drug-eluting stents not only repress proliferation and migration of vascular smooth muscle and endothelial cells, but also can elicit thrombosis.
Despite the reduction in restenosis rate by drug-eluting stents with respect to bare-metal stents, similar rates of overall mortality and myocardial infarction exist for treated patients whatever the stent type during long-term follow-up. However, careful selection of stent type according to patient and lesion features may optimize the therapy efficiency.
Drug-eluting stents delay healing and impair endothelialization observed with intravascular ultrasonography, as drugs target both smooth muscle and endothelial cells [783]. Furthermore, drugs released from the polymer favor thrombogenesis, as they impede the generation of thrombus inhibitor, i.e., the endothelium.10 Early and late stent thromboses occur with similar frequency with both stent types, but very late stent thrombosis characterizes drug-eluting stents. Antiplatelet therapy duration is thus longer for drug-eluting stents.
Endothelium-like tissue-coated stents are contrived to impede the consequences of damaged endothelium during stent implantation. Endotheliocytes are fragile and do not adhere strongly to the stent material, which bears very large deformations, in particular during stent implantation. Genetically engineered chondrocytes, which have the main endotheliocyte functions and better adhesion properties than endotheliocytes, can form a stent coating.
To support reendothelialization, stents can also deliver growth factors and chemoattractants of endothelial progenitors using marker antibodies, but patients with cardiovascular diseases often have few and/or dysfunctional endothelial progenitors [785]. However, nanomatrix that mimics endothelial surface with cell adhesion molecules and nitric oxide sources aims at recruiting endothelial progenitors.
9.4.3 Depot Stents
Depot stent is a drug-eluting stent with micrometric drug containers with given spatiotemporal release kinetics and programmable drug release. Unlike DESs that have their entire surface coated, depot stent contains a set of tiny drug reservoirs, thereby reducing the contact surface between the polymer and arterial wall that may favor inflammation and thrombosis.
The polymeric matrix and drugs fill orifices (diameter/strut width ratio 0.5), either closed-end holes (depth 0.5 strut thickness) or microchannels open at both ends. In the latter case, different types of drugs can be stored in the polymer, such as an endothelial cicatrizant in the channel part close to the wall and an anticoagulant in the part adjacent to the arterial lumen. However, such reservoirs can weaken the stent structure.
Openings can evenly spread over the entire stent surface or localize either in connectors or in bars or apices of stent rings bridged by connectors. The spacing between two neighboring reservoirs equals 150 μm. The number of containers determine the drug dose that can be stored, hence fully replacing that in the stent coating (thickness 5–15 μm).
With respect to stent without orifices, blind-hole reservoirs on connectors or ring bars affect neither the radial strength nor plastic strain, but reduce the fatigue safety factor [786]. Blind-hole containers evenly spread on the entire stent or only located in ring apices alter both the radial strength and plastic strain and lower the fatigue safety factor to a greater extent than those situated on connectors or ring bars. Throughhole reservoirs evenly spread on the entire stent or on apices degrade further stent resistance to mechanical stress. Through-hole containers on connectors or ring bars that have the maximal drug storage capacity may kept sufficient mechanical integrity [786].
Although drug-eluting, metallic stents have reduced in-stent restenosis and thrombosis rates with respect to previous generations of stents, the permanent presence of a metallic foreign body within the artery impair the vasomotor function and can favor vascular inflammation (Table 9.3). Furthermore, these stents disturb vascular healing, are responsible for side-branch jailing, and preclude late lumen enlargement, noninvasive imaging, and possible surgical revascularization of stented segments.
Table 9.3
Comparison of bioresorbable scaffolds (BRS) with other percutaneous intervention devices. (Source: [787])
Event | BA | BMS | DES | BRS |
---|---|---|---|---|
Acute occlusion | – | + | + | + |
Acute recoil | – | + | + | + |
Acute thrombosis | – | – | – | – |
Subacute thrombosis | ± | – | – | – |
Late thrombosis | + | – | – | ± |
Very late thrombosis | + | ± | ![]() | +/? |
Intimal hyperplasia | – | – | ± | + |
Constrictive remodeling | – | + | + | + |
Expansive remodeling | + | – | – | + |
Restoration of vasomotion | + | – | – | + |
Late luminal enlargement | + | – | – | + |
Biodegradable (BP) and permanent (PP) polymer coating on rapamycin-eluting stents have quasi-equivalent efficacy that is greater than that of the polymer-free (PF) stent without differences in safety [791].
9.4.4 Resorbable Stents (Scaffolds)
Biodegradable arterial (vascular) stents (BAS [BVS]), or bioresorbable scaffolds (BRS), represent the present step of conceiving, designing, optimizing, and manufacturing stents that deliver drug and disappear when they become useless (Table 9.4). They hence restore physiological strain and stress fields and mechanotransduction capability as well as obviate long-term anticoagulant therapy.
Table 9.4
Features of resorbable scaffolds, which are self-expanding or deploy upon heat or balloon inflation (Source: [787]). Metallic bioresorbable stents include drug-eluting magnesium-alloy scaffolds. Biodegradable polymers with variable surface properties are constituted of polyethylene glycol compounds attached to polylactic acid blocks in various types of combinations. Everolimus is a derivative of sirolimus. both inhibit target of rapamycin (TOR). Myolimus is a macrocyclic lactone and also a TOR inhibitor
Strut and coating material | Metallic (magnesium alloy) Polymeric (PDLLA, PLLA, PLAS, PTDPC, SA–AA) |
---|---|
Strut thickness | Metallic: 125–150 μm |
Polymeric: 150–230 μm | |
Eluted drug | Everolimus, myolimus, sirolimus, paclitaxel |
Bioresorbable vascular scaffolds ware first implanted in patients with stable or unstable angina and silent ischemia. Second-generation resorbable arterial scaffolds are now deployed in coronary arteries upon acute ST-segment elevation myocardial infarctions (STEMI) [788, 789]. Percutaneous coronary revascularization is currently the first choice treatment for patients with, as it is better than thrombolysis. Yet, stent placement in acutely thrombotic lesions often causes late stent malapposition and stent thrombosis because of thrombus sequestration between struts and the arterial wall. Multislice coronary computed tomography (MSCT) as well as invasive coronary angiography, intravascular ultrasonography (IVUS), optical coherence tomography imaging (OCT), and coronary vasomotor testing check quality of implantation and absence of complications, in addition to the follow-up.
Bioresorbable scaffolds have a greater conformability and flexibility than conventional stents, hence lowering scaffold strut malapposition as well as alterations of the endothelium, mural stress field, and arterial geometry. However, thicker struts and crossing profile (scaffold-to-artery size ratio
% [
% for DES] [788]) impede stent crossing of sharp angles and its implantation in heavily calcified or tortuous arteries.


Metallic bioresorbable scaffolds have properties similar to those of conventional metallic stents (deliverability, good radial strength, and low recoil) in addition to retarded resorption. Magnesium-based alloys can be coated with by a composite two-layer film that controls both the corrosion rate of the magnesium alloy and rate of drug release.
Polymeric bioresorbable scaffolds are made of various types of polymers, each with their own chemical characteristics and resorption time [787]. Polylactic acid, or polylactide, is a thermoplastic aliphatic polyester. The degradation of polymeric devices can be optimized by combining crystalline and amorphous polymers.
A gradual resorption happens during the first 2 years after implantation, thereby restoring after a relatively long delay the arterial geometry and physiology, including vasomotion [790]. The duration of its degradation depends on the crystallization of the polymer and varies from 2 to 4 years. Magnesium is resorbed by corrosion in 2–12 months. Magnesium scaffolds are metabolized to chloride, oxide, sulfate, or phosphate salts. The by-product in the artery is hydroxyapatite that is eventually processed by macrophages [787].
A more extensive artery preparation is required before implantation of biodegradable scaffolds. Unlike drug-eluting stents, resorbable scaffolds are less amenable to postdilation because of the risk of strut fracture. Therefore, precise estimation of the vessel size is mandatory for selecting the proper scaffold size, a nontrivial task due to eventual vasoconstriction and thrombi [790].
Deposition of a uniform fibrous neointimal layer may be associated with a late lumen enlargement [790]. On the other hand, resorbable scaffolds have downsides. Early scaffold thrombosis can occur. Despite good radial strength and low recoil, acute recoil of polymeric biodegradable stents can be observed [790]. In addition, polymeric devices that have a limited stretchability can break when they are overdilated.
Due to thicker struts (150–230 μm [DES: 80–140 μm]) and larger wall surface coverage than drug-eluting stents, resorbable arterial scaffolds can entrap and squeeze more thrombi and debris between the scaffold and arterial wall, in addition to higher risk of side-branch occlusion.
Analysis of drug-eluting bioresorbable arterial scaffolds in ST-segment elevation myocardial infarction shows a mean strut malapposition equal to
%, a mean incomplete scaffold apposition area
mm
, a mean intraluminal defect area to
mm
, and a mean percentage malapposed struts per patient to
% [788]. In acute ST-segment elevation myocardial infarction, implantation of bioresorbable arterial scaffolds causes acute scaffold recoil in 9.7 % patients, rate of scaffold strut malapposition in 1.1 %, and small and clinically silent edge dissections in 38 % [789]. Complete bioresorption takes at least 2 years after implantation.






9.4.5 Valvate Stents for Heart Valve Replacements
Aortic valves can be percutaneously replaced by valve-containing stents in the beating heart under image guidance, without strong damage of the aortic wall and obstruction of coronary ostia. First, percutaneous aortic valvuloplasty was proposed using balloon-expandable stents [792, 793]. Catheterized implantation uses a valve prosthesis that can be sutured into an expandable stent and keep its property after crimping and re-expansion. Bovine jugular venous valves are sutured inside the stent [794]. The valve-stent assembly is deployed by balloon inflation. Due to heavy periodic loading, self-expanding stents are preferred to balloon-expandable stents [795]. A pulmonary metal stent with a valve was very recently implanted using a catheter-based method in a child with a congenital heart defect.11
Percutaneous aortic valve implantation can be proposed in old patients with severe aortic stenosis, particularly patients judged inoperable or at high risk for surgical aortic valve replacement [796]. However, the global failure rate equals 33% with 25% periprocedural deaths. Moreover, vascular injury at the access site using femoral or iliac arteries can cause death. Periprosthetic regurgitation occurs in at least 10% of the cases. An atrioventricular block can necessitate pacemaker implantation. An alternative approach, like the transapical implantation, overcomes these drawbacks, especially incorrect implantation of the prosthesis [797].
9.4.6 Drawbacks of Medical Devices
Any implant device may induce several biological and mechanical disturbances when device design is not appropriate and its rheology does not match the wall properties. However, the device material is selected to avoid sensitization, cytotoxicity, and carcinogenicity.
Hemolysis occurs when a part of the device is located in the flow core.
The intact and healthy endothelium possesses an antithrombotic property, hence supporting normal blood flow by separating platelets for the extracellular matrix. Moreover, endotheliocytes release nitric oxide and prostacyclin, two soluble inhibitors of platelet activation. They also secrete high concentrations of ectonucleotide pyrophosphatase–phosphodiesterase ENPP1 that rapidly hydrolyzes extracellular nucleotides such as ADP. However, a medical device, like any implanted foreign body, provides a matrix for thrombus formation, especially when endothelial cells are damaged or removed during the intravascular implantation. In particular, stenting destroys large endothelial segments. A thrombogenic response occurs after platelet deposition and clotting activation. After the initial platelet adhesion, auto- and paracrine mediators (ADP, adrenaline, thromboxane-A2, and thrombin) amplify and sustain the platelet response, as they recruit circulating platelets to form a growing hemostatic plug.
Vessel-wall damage also generates an intimal cell proliferation and quick restenosis . Growth factors can be released from platelets and fibroblasts. An inflammation due to interactions between leukocytes and the device can occur once leukocytes and complement have been activated. Reactive oxygen and nitrogen species ( superoxide and peroxynitrite) and effectors (e.g., poly
ribose polymerase)12 participate in restenosis after vascular injury. In media and neointima, NOS2 is frequently overexpressed. The serum 3-nitrotyrosine-to-tyrosine ratio (protein Tyr nitration),13 an index of oxidative stress mainly resulting from neutrophil activation, is correlated with the narrowing of the lumen [799]. On the other hand, the NOS–NO–sGC axis attenuates intimal hyperplasia.

Finally, the medical device disturbs blood flow. Locally, the added interface rugosity caused by the implanted device can generate local flow separations. Moreover, the abrupt transition between native vessel and stented segment induces a compliance mismatch. By means of wave reflection, it can remotely affect blood flow.
9.4.7 Design of Medical Devices
Computer-aided manufacturing allows one to test easily diverse device geometries, structures, and material properties, with an important requirement: the material matching of the mechanical properties of the biological tissues. It needs to model accurately the physical processes at the interface, because the interface between the implants and the biological tissues (blood and vascular wall) is the focus of desirable and undesirable interactions. Moreover, it must be adaptable to unsteady loading induced by cardiac contractions.
9.5 Stem Cell-Based Regenerative Therapy
The adult heart experiences a cardiomyocyte turnover and hence cannot be considered as a fully terminally differentiated organ. However, the heart can regenerate only during the first week after birth. After this short postnatal period, the majority of cardiomyocytes permanently exit the cell division cycle. The renewal by cell division in the human myocardium occurs at a very low rate (1 and 0.45 % annually at the age of 25 and 75 year, respectively) [800]. After ischemia, the renewal reserve is unable to rescue a failing myocardium.
At birth, the placental-to-pulmonary circulation switch inaugurates cardiac aerobic metabolism. In cardiomyocytes, during this transition, the mitochondrial genesis increases and its intracellular structure remodels under control of mitofusins Mfn1 and Mfn2 [801].
During embryo- and fetogenesis, relatively hypoxic cardiomyocytes rapidly proliferate and use anaerobic glycolysis as a main source of energy:
In adult cardiomyocytes, the oxidative phosphorylation is the energy source. The mitochondrial tricarboxylic (or citric) acid cycle coupled to oxidative phosphorylation by the electron transport chain associated with ATP synthase at the inner mitochondrial membrane via NADH produces 18-times more ATP than cytoplasmic glycolysis. AcetylCoA is provided by glucose and amino and fatty acid metabolisms:

(P
: inorganic phosphate or free phosphate ion).


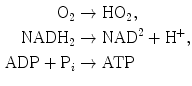

In mouse cardiac mitochondria from postnatal day 7 with respect to newborn mice, DNA-content, crista-density, and aerobic-respiration enzyme levels increase. Mitochondrial ROS are generated as a consequence of electron leak by the electron transport chain.
The transition to an oxygen-rich postnatal environment and hence to an aerobic metabolism engenders DNA damage that causes cardiomyocyte cell-cycle arrest and hence stops proliferation [802]. Concentrations of reactive oxygen species, oxidative DNA damage, and DNA damage-response markers significantly rise in the heart during the first postnatal week. Postnatal hypoxemia, diminished mitochondrialdependent oxidative stress and ROS scavenging, or DNA damage-response inhibition prolong the postnatal cardiomyocyte proliferative time window. On the other hand, hyperoxemia and ROS generation shorten it.
As the heart has a reduced repair capacity, early reperfusion of occluded artery after acute myocardial infarction raises the long-term prognosis. However, it does not prevent chronic myocardial dysfunction and development of heart failure. Early after myocardial infarction, therapy is primarily cardioprotective, that is, salvaging the jeopardized myocardium and preventing adverse remodeling.
At a later stage, the goal is cardiorestorative, that is, aimed at reversing maladaptive remodeling and improving contractility using activation of resident cardiac progenitors and stimulation cardiomyocyte entry in the cell division cycle as well as increasing neovascularization and decreasing scar burden [803].
Transplantation of stem or progenitor cells aimed at improving revascularization of ischemic regions, reducing inflammation, and regenerating injured cardiac tissue can complement current therapy (Vol. 5, Chap. 11. Tissue Development, Repair, and Remodeling). This strategy relies on optimization of the progenitor cell type and engraftment site that engender the best possible tissue integration, thereby avoiding immune rejection of engrafted cells and excluding tumorigenic precursors.
During acute myocardial infarction, two peaks of VEGF release can be detected: early (24–48 h) and late (
day). They can influence stem cell transplantation efficiency.
< div class='tao-gold-member'>

Only gold members can continue reading. Log In or Register a > to continue
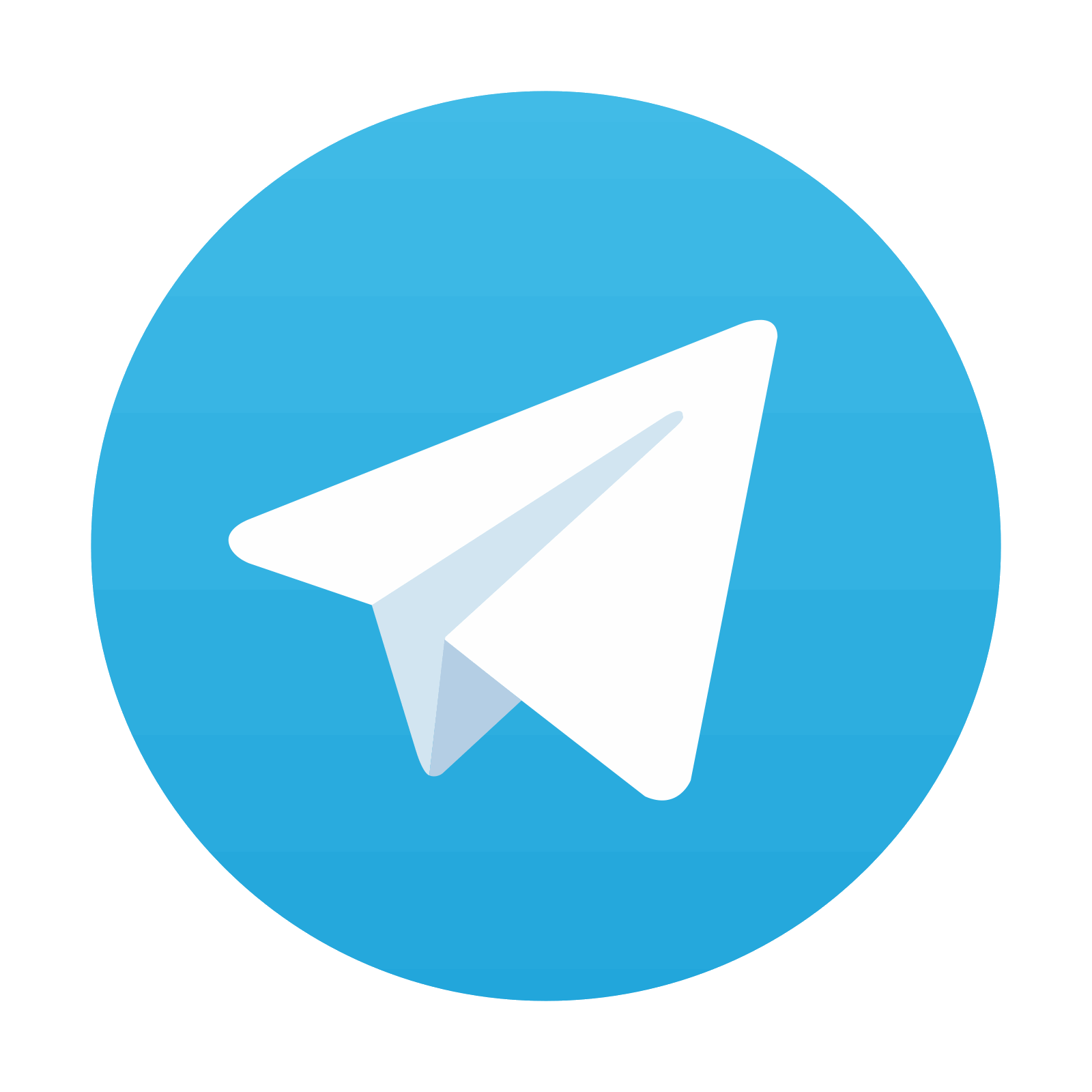
Stay updated, free articles. Join our Telegram channel
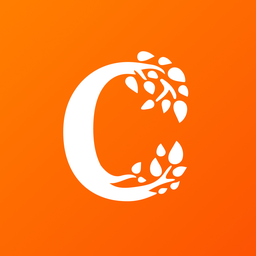
Full access? Get Clinical Tree
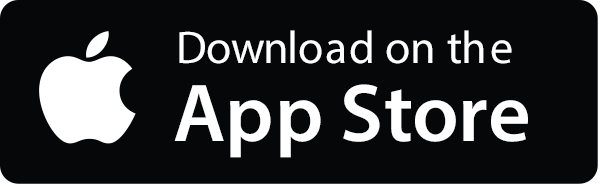
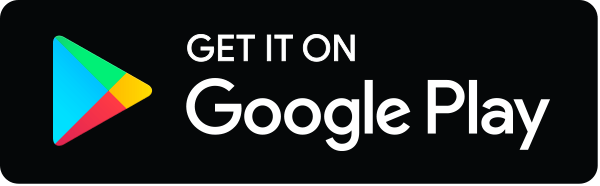