Ventricular pressure stroke volume curve. With a decrease in systemic venous return, the ventricle moves from position C to B, diastolic volume and therefore pressure decrease while stroke volume remains unchanged. With a further decrease in systemic venous return (B to A), ventricular diastolic volume and pressure fall further and stroke volume decreases. Conversely, moving from position A to B with volume administration for example increases stroke volume; however, additional volume (B to C) increases diastolic volume and pressure but stroke volume does not increase
The Effects of Respiration on Right Ventricular Afterload
Respiration affects pulmonary vascular resistance (PVR) by altering blood pH, alveolar oxygen tension, and lung volumes. Respiratory and metabolic alkalosis induces pulmonary vascular vasodilation, whereas acidosis causes vasoconstriction.
Respiration also affects PVR by altering lung volumes. This cardiopulmonary interaction is not mediated by changes in ITP per se, but rather is a function of the alveolar Ptm, regardless of the mode of ventilation, and respiratory system compliance. Alveolar vessels lie within the septa, which separate adjacent alveoli. Alveolar pressure is the surrounding pressure for these vessels. Extra-alveolar vessels are located in the interstitium and are exposed to intrapleural or ITP. Because alveolar and extra-alveolar vessels are in series, the resistance provided by each are additive. FRC is the lung volume from which normal tidal volume breathing occurs. PVR is lowest near the FRC and increases at both high- and low-lung volumes albeit for different reasons.
At low-lung volumes, the radial traction provided by the pulmonary interstitium diminishes, leading to a decrease in the cross-sectional area of the extra-alveolar vessel. In addition, low end-expiratory lung volumes and alveoli collapse lead to hypoxic pulmonary vasoconstriction, and the resistance of extra-alveolar vessels increases further. Meanwhile, the Ptm of alveolar vessels increases, the vessels distend, and resistance falls because the alveolar Ptm has decreased. Nonetheless, the net effect is for PVR to increase at low-lung volumes.
As lung volumes rise well above FRC, PVR increases. Large tidal volumes or tidal volumes superimposed on an elevated FRC significantly increase PVR. With large lung volumes, overdistended alveoli compress interalveolar vessels, decreasing the Ptm for the interalveolar vessel and increasing PVR. With PPV, the interstitial pressure is positive, decreasing the Ptm for the extra-alveolar vessels as well, contributing to PPV-induced increases in PVR. In other words, during PPV, alveolar and intrapleural pressures are positive during inspiration and expiration and resistance is elevated in both alveolar and extra-alveolar vessels throughout the respiratory cycle. This is in contrast to an increase in lung volume due to negative pressure ventilation where interstitial pressure is negative. Despite the fact that alveolar recruitment improves oxygenation thereby releasing hypoxic pulmonary vasoconstriction of extra-alveolar vessels, and an increase in lung volume increases the radial traction and cross-sectional area of the extra-alveolar vessels, the net effect of large lung volumes is to increase PVR.
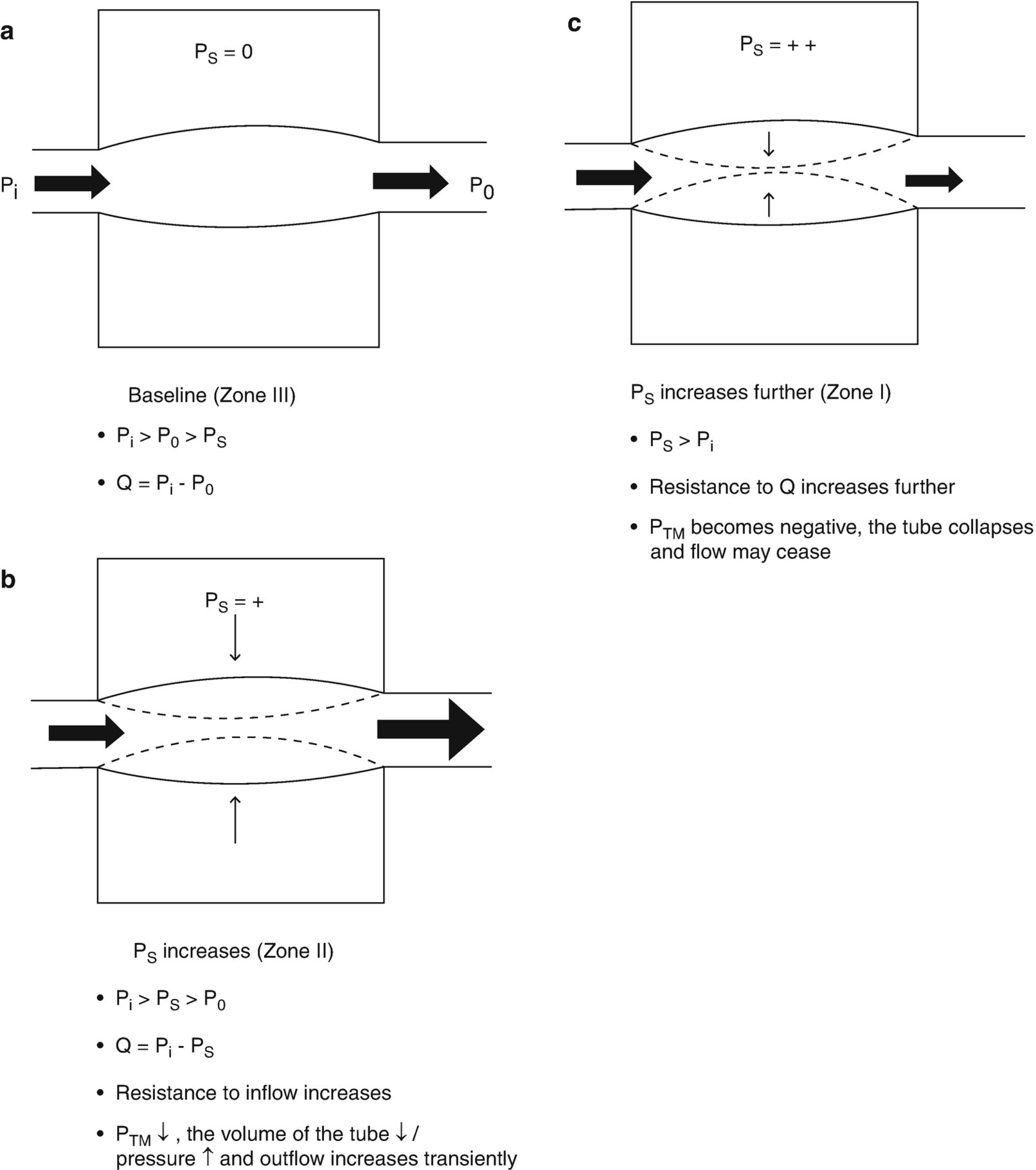
The relationship between lung volume, pulmonary vascular hydrostatic pressure, and pulmonary vascular resistance in a patient receiving positive pressure ventilation for acute respiratory distress syndrome. Ps, surrounding (alveolar) pressure for the interalveolar vessel; Pi and Po, inlet and outlet pressure for the interalveolar vessel; Q, flow; Ptm, transmural pressure. Condition A: Base of the lung where hydrostatic pressure is at its greatest and Ps is at its lowest (collapsed lung): Pi and Po are greater than Ps, and the alveolar vessel is widely patent; zone III conditions; Q = Pi – Po. Condition B: Proceeding from the base to the apex of the lung. Vascular hydrostatic pressure has fallen due to the effects of gravity, and Ps has increased as airway pressure distends alveoli to a greater extent in the less gravity-dependent portions of the lung. Pi > Ps > Po, resistance to flow has increased as the Ptm for the alveolar vessel has decreased and the vessel is partially compressed; Q = Pi – Ps; zone II conditions. Condition C: Apex of the lung where vascular hydrostatic pressures are at their minimum and alveoli are over distended. Ps > Pi, the Ptm for the alveolar vessel becomes negative, the vessel collapses, and Q ceases; Zone I conditions
RV Performance and Ventricular Interaction
An important determinant of the impact of PPV -induced increases in RV afterload on cardiopulmonary function is RV systolic function. The RV contracts by 3 mechanisms: inward movement of the free wall, which produces a bellow-like effect; contraction of the longitudinal fibers, which shortens the long axis and draws the tricuspid annulus toward the apex; and traction on the free wall at the points of attachment secondary to left ventricular (LV) contraction (i.e., systolic ventricular interaction – discussed below) [6]. With a progressive increase in RV afterload, compensatory ventricular hypertrophy maintains ventricular arterial coupling and stroke volume is preserved [7]. However, even a modest acute increase in afterload causes stroke volume from the unprimed RV to decrease, which is much less responsive to increases in preload and much more sensitive to increases in afterload than the LV.
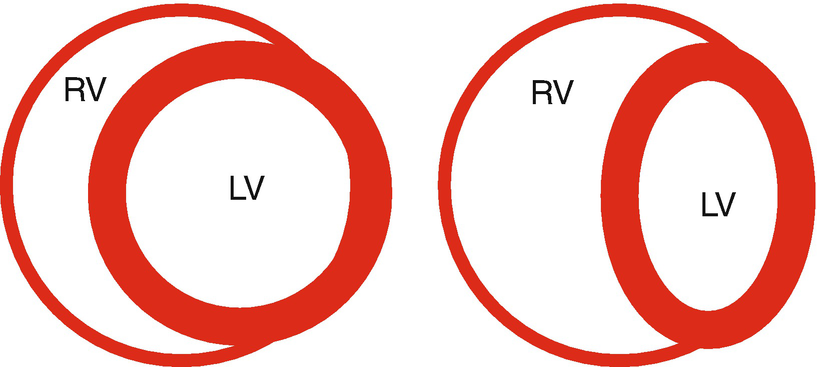
Ventricular interdependence. Cartoon of a parasternal short-axis view of the left and right ventricles (LV, RV). Under normal conditions, the interventricular septum is oriented such that in the short axis the LV is circular. Under conditions when the pressure in the RV is elevated, the interventricular septum is displaced to the left, decreasing the effective compliance of the LV. Without an increase in the LV diastolic transmural pressure, LV filling decreases
RV afterload-induced systolic dysfunction causes ventricular volume and therefore pressure to be elevated throughout the cardiac cycle, decreasing if not eliminating the normal transseptal pressure gradient (Fig. 13.3). Under normal conditions, LV diastolic pressure is greater than right causing the interventricular septum (IVS) to bow into the RV (Fig. 13.3). With RV diastolic hypertension, the IVS occupies a more neutral position between the two ventricles (Fig. 13.3). If RV diastolic pressures were to rise above left, the septum would actually bow into the LV. In either case, the LV becomes restrained not only by RV pressure and the deviated septum but also its free wall becomes constrained by the pericardium and potentially lung. These factors decrease the effective compliance of the LV. Even though LV diastolic pressure is elevated, intrapericardial pressure has risen to a greater extent, and the net effect is a reduced LV diastolic Ptm and LV filling. This phenomenon is known as diastolic ventricular interdependence.
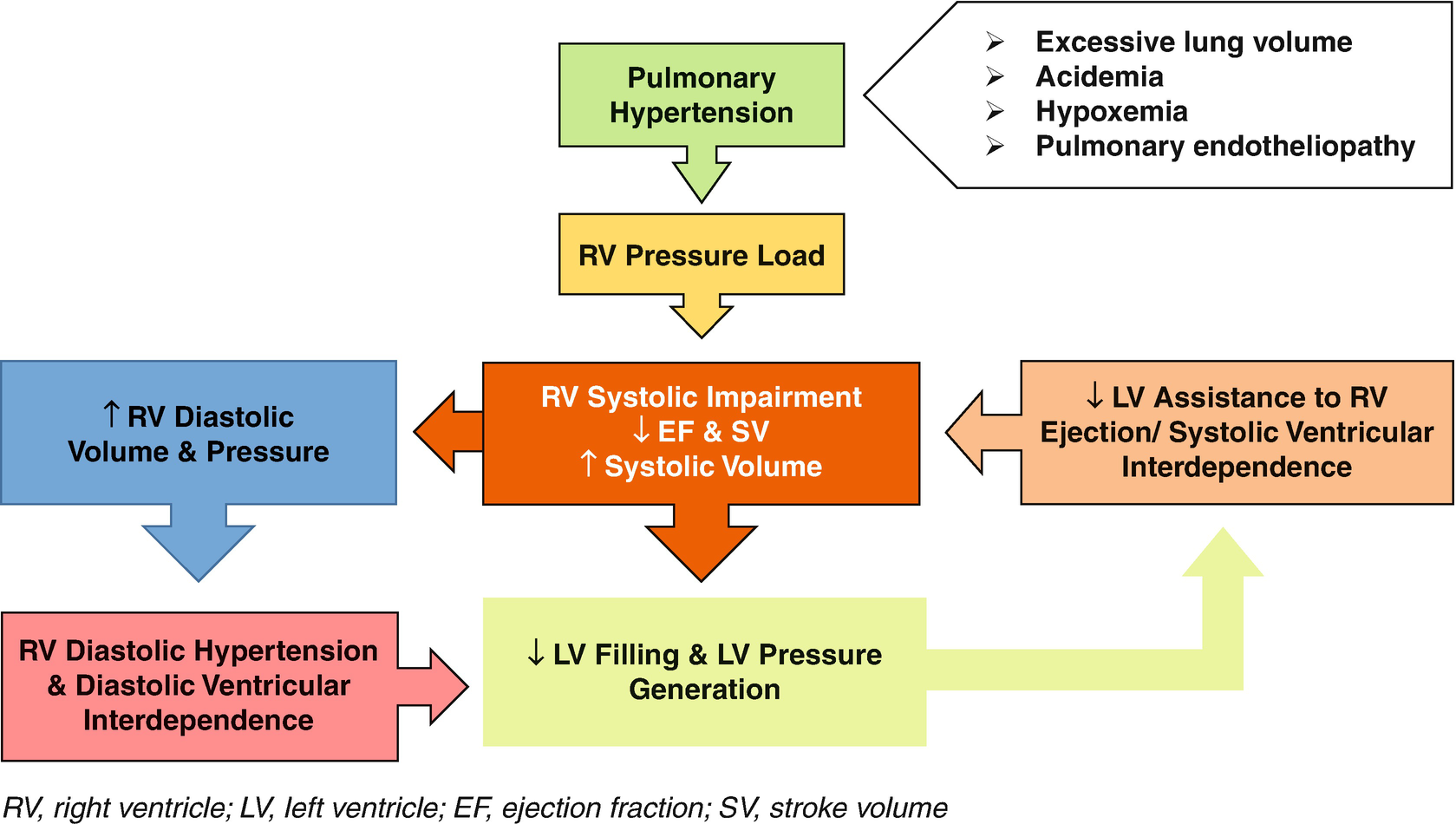
Pathophysiology of low cardiac output in pulmonary arterial hypertension. RV right ventricle, LV left ventricle, EF ejection fraction, SV stroke volume
Respiratory Mechanics
Most commonly the decrease in compliance, or increase in elastance, of the respiratory system in ARDS has been attributed to changes in lung compliance. However, it is important to consider changes in chest wall compliance when implementing PPV to improve oxygenation and just as importantly when considering and discussing the impact of PPV on RV loading conditions and output.
The respiratory system includes the lung and chest wall and the overall mechanical behavior depends on the mechanical characteristics of its components and their interactions. The chest wall, consisting of the thoracic cage and diaphragm (the latter mechanically links the abdominal cavity to the thorax), and lungs are in series and may be expressed as follows when airway resistance is minimal: Paw = Ptp + Ppl and Etot = EL + Ecw, where Paw is static airway pressure, Ptp is transpulmonary pressure, Ppl is pleural pressure, Etot is total respiratory system elastance (elastance is inversely related to compliance), EL is lung elastance, and Ecw is chest wall elastance [8]. By rearranging this equation, it follows that: Ppl = Paw × Ecw/Etot, which enables us to consider the impact of changes in lung and chest wall elastance on Ppl (ITP) and therefore the impact of Paw on RV loading conditions under a variety of conditions.
For a given Paw, as Ecw rises Ppl increases, increasing ITP and RA pressure and potentially decreasing systemic venous return, while decreasing the RV diastolic Ptm, contributing to a decrease in RV filling. For the same Paw, as Ppl rises the Ptp decreases, decreasing end-expiratory lung volume and tidal volume, with an indeterminate effect on RV afterload (derecruitment and hypoxic pulmonary vasoconstriction versus decreasing the extent of alveolar overdistension and the proportion of lung regions under zone I and II conditions). Conversely, for a given Paw, as EL increases, the transmission of airway pressure and thus Ppl decreases.
Chest wall elastance may be elevated due to a number of factors, including chest wall edema and pleural effusions, as well as factors responsible for increasing abdominal pressure, such as obesity, ascites, and visceromegaly. The importance of considering Ecw in the management of ARDS cannot be overstated, as it has a profound impact on respiratory and cardiovascular function and at least in adults may impact outcomes [8].
The Effects of Respiration on Left Ventricular Afterload
While the primary impact of PPV on cardiovascular function in patients with ARDS is on RV loading conditions, other factors may come into play that adversely impact LV function (e.g., underlying LV dysfunction, sepsis-mediated ventricular dysfunction). Thus, an understanding of the impact of respiration on LV function is relevant to the management of the critically ill patient with ARDS [2]. Just as changes in ITP affect the return of blood from the periphery to the heart, so too does it affect the egress of blood from the thoracic to extrathoracic arterial system.
During spontaneous respiration, the fall in ITP increases the Ptm and therefore volume for the thoracic arterial system. As a result, the pressure within these structures decreases relative to the extrathoracic arterial system and LV afterload increases. If the fall in ITP occurs during ventricular diastole, antegrade flow runoff decreases, resulting in an increase in thoracic arterial blood volume and an increase in the inertial forces opposing ejection during the following systole. A fall in ITP during ventricular systole decreases LV ejection and stroke volume and the egress of blood from the thorax. As LV systolic function wanes or as ITP becomes more negative, the adverse impact of respiration on LV afterload increases. With PPV, the decrease in Ptm for the thoracic arterial system increases the pressure within these structures (“reverse pulsus paradoxus”) creating a waterfall-like effect, driving blood into the extrathoracic compartment.
With impaired LV systolic function, the rise in pulmonary venous pressure will markedly enhance the rate and extent of extravascular lung water (EVLW) formation, necessitating even greater positive airway pressure. However, the elevation in pulmonary venous pressure significantly reduces the propensity to develop lung regions under zone I and II conditions. Meanwhile, because the afterload for the RV is elevated, it should be operating on the flat portion of its pressure stroke volume curve, rendering it less sensitive to decreases in systemic venous return. Therefore, PPV should have a significant beneficial impact on cardiopulmonary function in these circumstances.
The Acute Respiratory Distress Syndrome
Pathophysiological Processes
ARDS results from inflammation caused by pulmonary and/or extrapulmonary insults, most commonly pneumonia and sepsis, respectively, which transforms the pulmonary endothelial cell phenotype into a prothrombotic and proinflammatory state. Activated neutrophils are sequestered within the pulmonary vasculature, where they cause endothelial and alveolar epithelial damage, leading to a marked increase in vascular permeability and the development of interstitial and alveolar edema, as well as allowing for the extravasation of plasma proteins and the formation of alveolar hyaline membranes. Injury to type II alveolar epithelial cells type decreases surfactant production, which is compounded by surfactant inactivation by extravasated plasma proteins. The decrease in surfactant activity increases alveolar surface tension forces, promoting alveolar collapse.
While the increase in air–blood barrier permeability is evenly distributed, and EVLW is diffusely increased, a vertical, gravitational gradient exists for lung densities and EVLW formation [9]. The increase in lung weight exaggerates the normal compressive gravitational forces present in lung parenchyma, leading to the formation of nonaerated tissue in gravity-dependent regions of the lung. Lung tissues in nondependent regions of the lung are well aerated with near-normal mechanical characteristics [9].
Pulmonary hypertension is invariably present to a varying degree in patients with ARDS [10]. The are several factors responsible for the development of pulmonary hypertension including those related to lung volume described previously and include a shift in the pulmonary endothelial cell phenotype to a prothrombotic and proinflammatory state, leading to microvascular occlusion by thrombi and cells (neutrophils and platelets) and endothelial injury and dysfunction, leading to an imbalance between vasodilating and vasoconstricting mediators.
The primary gas exchange abnormality in ARDS is impaired oxygenation due to alveolar collapse. Studies of adult patients with ARDS have demonstrated that the primary abnormality responsible for arterial hypoxemia is pulmonary shunt, with only a small portion of lung units demonstrating low ventilation to perfusion (V/Q) ratios [11]. As with other lines of investigation, diffusion abnormalities do not contribute to impaired oxygenation [12]. Hypoxic pulmonary vasoconstriction limits perfusion to non- or poorly ventilated alveoli, improving the relationship between ventilation and perfusion and oxygenation. An additional factor that may contribute to arterial hypoxemia is the presence of a patent foramen ovale and right-to-left atrial shunting. Areas of high ventilation to perfusion ratios contribute to impaired gas exchange in ARDS by wasting ventilation and impairing CO2 clearance, and result from those factors that lead to alveolar overdistension and a decrease in pulmonary perfusion. The presence of right-to-left atrial shunting and dead-space ventilation not only impact the acute management of but have been shown to be of prognostic significance in adult patients with ARDS [13–15].
PPV-Induced Cardiovascular Dysfunction in ARDS
Despite the use of lung-protective ventilation for ARDS, PPV may induce significant heart–lung interactions that adversely impact gas exchange, RV loading conditions, CO and ultimately systemic DO2 [16]. PPV may compromise RV output predominantly as a result of an increase in ITP and decrease in systemic venous return and RV filling, increase in PVR and impaired RV systolic performance, or a combination of both [16].
More than 60% of adult patients with ARDS experience hemodynamic failure, necessitating vasoactive support [17, 18]. ARDS is frequently associated with RV dysfunction, the most severe form of which is acute cor pulmonale, which occurs in 25–30% of adult patients with ARDS [19, 20]. Further, RV dysfunction has been shown to be independently associated with a higher risk of death in these patients [21, 22].
PPV may decrease RV filling by increasing RA pressure and decreasing the pressure gradient for systemic venous return. The extent to which this occurs depends on the adequacy of circulatory reflexes to elevate the Pms, where the RV is positioned on its pressure stroke volume curve and the degree to which airway pressure is transmitted to intrathoracic structures, the latter of which is a function of respiratory system elastance. In any case, a greater RV diastolic Ptm is needed to maintain RV filling, as PPV reduces the effective compliance of the RV.
RV output may be decreased due to primarily an increase in afterload, resulting from PPV-induced alveolar overdistension. As discussed, during PPV the non-gravity-dependent region of the lung is well aerated and possesses near-normal compliance and thus receives a disproportionate amount of the airway pressure, resulting in alveolar overdistension (Fig. 13.2) [9]. Blood flow in this region is limited due to the effects of gravity on pulmonary perfusion, which is compounded by the factors that limit RV output such as decrease in RV preload reserve, pulmonary endothelial dysfunction, and, in severe sepsis, cytokine-mediated cardiomyocyte dysfunction (Fig. 13.2) [23, 24]. Pulmonary vascular resistance increases to the extent that zone I and II conditions are created in the lung, which depends on alveolar and interalveolar vessel pressures.
A limitation of preload and increase in afterload may combine to decrease RV output. With an increase in afterload, a compensatory increase in preload is needed to maintain stroke volume, which may be limited for the reasons discussed [25]. Vieillard-Baron and colleagues described this finding in their study of adult patients with ARDS using echocardiography with Doppler to evaluate beat-to-beat inflow and outflow and ventricular dimensions throughout the respiratory cycle [25]. They demonstrated inspiratory reductions in RV fractional area contraction associated with a significant increase in RV end-systolic dimensions, while RV end-diastolic area remained unchanged. They concluded that this likely reflects a relative decrease in RV preload because an increase in afterload and end-systolic volume should produce a corresponding (compensatory) increase in preload.
The incidence and severity of PPV -induced cardiovascular dysfunction in pediatric ARDS are unknown. A timely and objective assessment of cardiovascular function is essential for optimizing the management of the pediatric patient with ARDS.
Assessment of Cardiovascular Function and Tissue Oxygenation
Hemodynamic insufficiency: assessment and treatment strategies
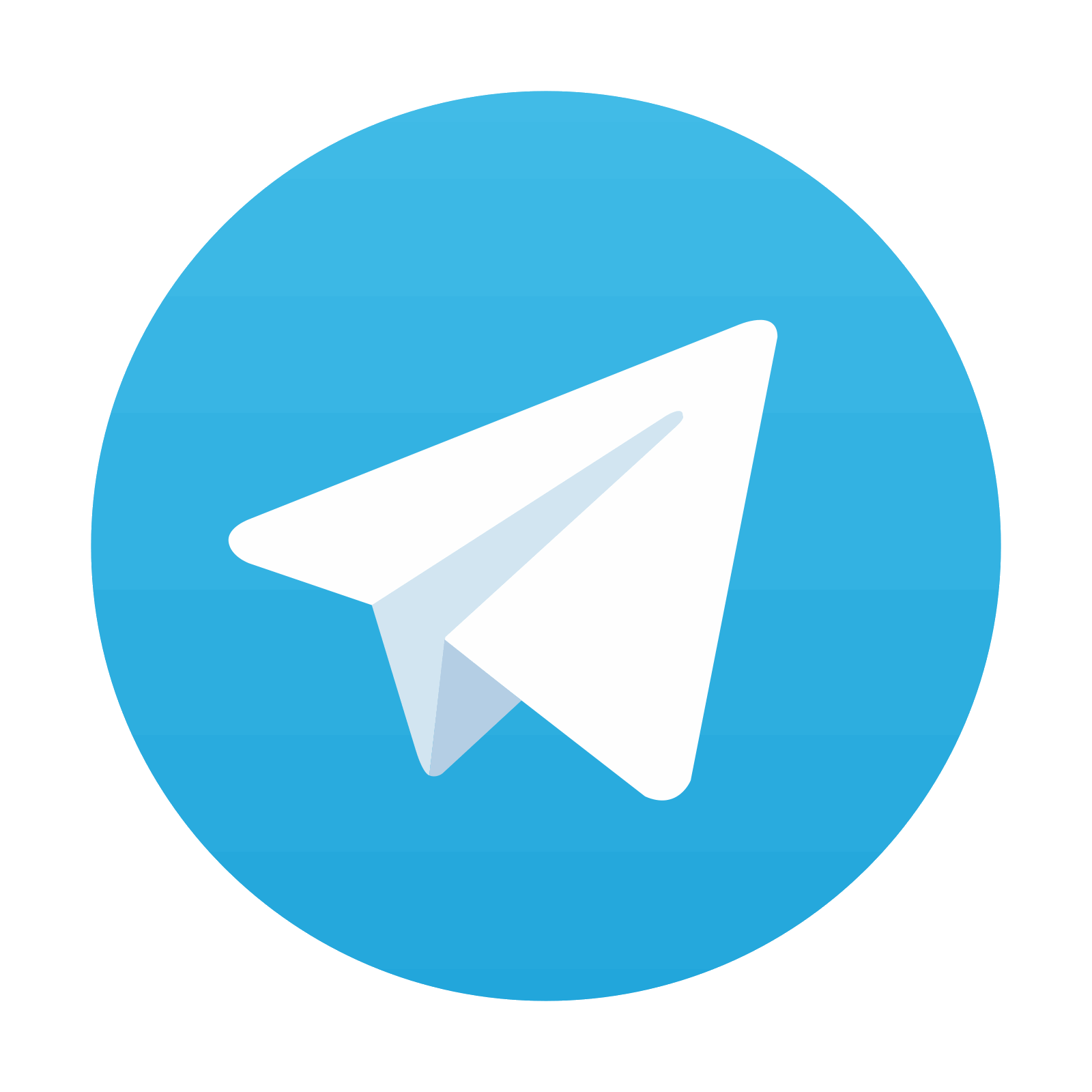
Stay updated, free articles. Join our Telegram channel
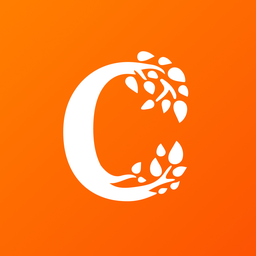
Full access? Get Clinical Tree
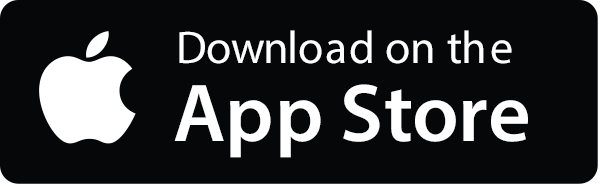
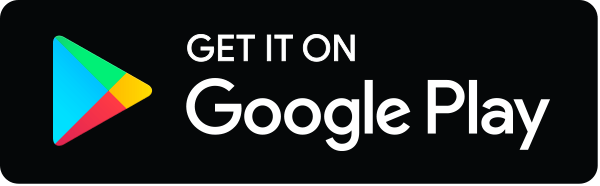