Background
Changes in vascular and myocardial structure and function have been demonstrated in obese children, but limited data are available on how these changes are related. The aims of this study were to investigate vascular and myocardial changes in obese children with lipid abnormalities and to study the interactions between vascular and myocardial parameters.
Methods
A cross-sectional, prospective observational study was conducted. Twenty-one obese and 27 normal-weight controls aged 14 ± 2 years participated. Cardiac assessment included geometric parameters and myocardial deformation (strain and strain rate) analysis by color tissue Doppler and speckle-tracking echocardiography. Vascular assessment included carotid intima-media thickness, flow-mediated dilatation, pulse-wave velocity, and other stiffness measures of the aorta and carotid artery, as well as noninvasive estimation of arterial elastance and left ventricular (LV) end-systolic elastance.
Results
Obese children compared with controls had lower color tissue Doppler–derived LV systolic radial strain values (45 ± 11% vs 56 ± 12%, P = .002), lower speckle-tracking echocardiography–derived LV systolic longitudinal strain values (−18 ± 2% vs −21 ± 2%, P < .001), and lower speckle-tracking echocardiography–derived LV early diastolic strain rate values (1.7 ± 0.3 vs 2.5 ± 0.4, P < .001). Carotid intima-media thickness was increased, pulse-wave velocity was faster, and arterial distension coefficients were lower in obese children. The ratio of arterial elastance to LV end-systolic elastance (a marker of ventricular-arterial coupling) was lower in obese children than controls (0.73 ± 0.32 vs 0.47 ± 0.15, P = .003). Changes in vascular parameters were correlated with changes in longitudinal myocardial deformation parameters.
Conclusions
Obese children with lipid abnormalities have reduced systolic and diastolic LV deformation characteristics, early vessel wall changes, and increased arterial stiffness. Abnormal ventricular-vascular interaction is suggested by these data and warrants further investigation.
The prevalence of obesity in adults and children is increasing worldwide. Because of its associated morbidities and effect on long-term outcomes, this is an important health care problem. Various studies have investigated the cardiovascular effects of childhood obesity, but reported results are sometimes conflicting. Vascular changes, including increased arterial stiffness, endothelial dysfunction, and subclinical atherosclerosis, have been demonstrated in different pediatric studies. In adults, similar vascular changes predict cardiovascular morbidity and mortality, but it is uncertain whether these data can be extrapolated to the pediatric group. Adult obesity can be associated with cardiac dysfunction, as indicated by an increased risk for heart failure and cardiomyopathy. This has not been shown in children, but recent studies in both children and adults have suggested that subtle systolic and diastolic functional abnormalities can be present in obese subjects with preserved ejection fractions. Using echocardiographic techniques such as tissue Doppler and myocardial deformation imaging (strain and strain rate imaging), changes in systolic and diastolic function have been described in both adults and children. Until now, most studies have focused on either vascular or myocardial effects, and few studies have integrated vascular and ventricular assessment.
The aim of this pilot study was to investigate the interaction between vascular and myocardial changes in a subgroup of obese children with lipid abnormalities, using a combined detailed vascular and ventricular evaluation. These children probably are a higher risk obese population, and we wanted to test the methodology first in this high-risk group before studying larger numbers of obese children.
Methods
Study Participants
We performed a cross-sectional, prospective observational study. Participants were recruited from the Lipid Clinic of the Hospital for Sick Children in Toronto, Ontario, Canada. Twenty-one overweight or obese children aged 10 to 18 years were included. Overweight was defined as a body mass index (BMI) between the 85th and 94th percentiles, and obesity was defined as BMI ≥ 95th percentile using Centers for Disease Control and Prevention growth charts. Because 20 of the 21 overweight and obese children had BMIs ≥ 95th percentile, we use the term “obese” to refer to the combined overweight and obese group. Apart from being obese, all children had lipid abnormalities, as they were referred for assessment and management to the pediatric lipid clinic. Twenty-seven sex-matched and age-matched normal weight controls (BMI < 85th percentile) were randomly selected from our normal control database of 170 healthy children who underwent extensive vascular and cardiac assessments. Children with familial hypercholesterolemia, antihypertensive medications, hemodynamically significant cardiac or renal disease, or systemic arterial hypertension not related to obesity were excluded from enrollment. The institutional research ethics board approved this study, and written informed consent was obtained from each participant and/or his or her parents before enrollment.
Clinical Evaluation and Family History
Data were collected for obese and control children regarding age, sex, height, weight, body surface area (BSA), BMI and BMI percentile, blood pressure, and resting heart rate.
Echocardiography and Offline Myocardial Deformation Analysis
Echocardiographic examinations were performed using a Vivid 7 ultrasound system (GE Medical Systems, Wauwatosa, WI). An anatomic and functional echocardiographic study was performed, as has been detailed elsewhere. Ventricular dimensions and left atrial dimensions were transformed into standard deviation scores ( Z scores) using institutional reference values based on BSA. Ejection fraction was calculated using the biplane Simpson’s method. Left ventricular (LV) mass was calculated using Devereux’s method and normalized to height 2.7 and BSA. End-systolic wall stress was calculated according to Mese et al. Pulsed-wave tissue Doppler velocities, grayscale images (parasternal short-axis images at the midventricular level and from the standard apical four-chamber views) for speckle-tracking echocardiography (STE) and color Doppler images (parasternal short-axis images at the midventricular level) for color tissue Doppler (CTD) deformation were acquired as previously described by our group. EchoPAC version 7.0 (GE Medical Systems) was used to obtain STE-derived longitudinal and circumferential strain and strain rate. We did not use STE for the assessment of radial strain, because we have previously found low reproducibility for radial strain and strain rate values by STE. Briefly, the endocardial border was manually traced at end-systole (starting at the mid septum for short axis and the basal septum from the apical four-chamber view). Tracking was automatically performed, and the analysis was accepted after visual inspection and when the software indicated adequate tracking. If tracking was suboptimal, the endocardial border was retraced. If satisfactory tracking was not accomplished within 5 min, the nontracking segments were excluded from analysis. Lagrangian radial strain and strain rate curves and circumferential strain curves from the short-axis view (six segments: anterior septum, anterior, lateral, posterior, inferior, and septum) and longitudinal strain curves from the apical four-chamber view (six segments: basal septum, mid septum, apical septum, apical lateral, mid lateral, and basal lateral) were obtained. Strain curves were visually inspected, and if the absolute strain value was equal to or less than an arbitrary value of 5% in a certain segment, the measurement for that particular segment was excluded from the analysis. The automated timing of aortic valve closure was used. End-systolic values for strain and peak systolic and early diastolic values for strain rate were measured. All offline measurements with EchoPAC were performed by a single observer (C.S.). Global strain was calculated as the average of the segments when three or more of six segments were measurable. Software Package for Echocardiographic Quantification Leuven version 4 (University of Leuven, Leuven, Belgium) was used to obtain CTD-derived radial strain and strain rate values. Briefly, the software allows the estimation of natural strain rate curves by measuring the spatial velocity gradient in a user-defined region of interest. The length of the region of interest was 8 mm. The region of interest was manually placed at the midmyocardial portion of the posterior wall of the short-axis image. An M-mode algorithm provided by the software was applied to track the region of interest throughout the cardiac cycle. Blood-pool pulsed-wave tissue Doppler traces of mitral valve inflow and aortic valve outflow were used to time cardiac events. Natural strain curves were obtained by integrating the mean strain rate profile over time and averaged for three to five cardiac cycles. Strain curves were visually inspected, and the same criteria for segment exclusion as for EchoPAC were used. Natural strain values were mathematically converted into Lagrangian strain values as previously described. Because the images allowed identification of the obese patients, we did not attempt to blind the observer to study group. No children were excluded from the study a priori because of poor echocardiographic windows or inability to perform offline analysis of myocardial deformation.
Intraobserver and interobserver variability of the CTD-derived radial strain and STE-derived longitudinal and circumferential strain measurements was evaluated in 10 randomly selected obese subjects. For intraobserver variability, the same observer remeasured the same images ≥2 weeks after the first assessment. For interobserver variability, deformation parameters were measured on the same images by two independent observers. The coefficient of variation (COV; the standard deviation of the difference of paired samples divided by the average of the paired samples) was calculated.
Vascular Assessment
All vascular measurements were performed by two experienced vascular sonographers in a quiet room after an overnight fast and 10 min of supine rest, using standardized protocols. Right-arm blood pressure was measured using an arm size-appropriate cuff as the average of three readings with an automated Dinamap sphygmomanometer (Critikon, Tampa, FL) and recorded along with resting heart rate. Carotid intima-media thickness (CIMT) was assessed using high-resolution B-mode imaging of the right and left carotid arteries using the Vivid 7 ultrasound system with a 12-MHz linear-array transducer. The mean CIMT was measured using an automatic edge detection algorithm (Carotid Analyzer for Research version 5.7.4, Vascular Tools; Medical Imaging Applications LLC, Coralville, IA), as previously described by our group. Endothelial function was studied by measuring flow-mediated dilatation (FMD), defined as the maximal percentage change in right brachial arterial diameter in response to reactive hyperemia, induced by inflating a blood pressure cuff to >200 mm Hg around the forearm for 5 min. High-resolution B-mode imaging was used to acquire longitudinal, electrocardiographically gated, end-diastolic images of the right brachial artery just above the antecubital fossa every 3 sec, and the brachial artery diameters were measured offline using the software automatic edge detection algorithm (Brachial Analyzer; Medical Imaging Applications LLC). Pulse-wave velocity (PWV) was assessed by sequentially recording electrocardiographically gated right carotid and right femoral artery waveforms using a high-fidelity micromanometer (SPC-301; Millar Instruments, Houston, TX). The wave transit time was determined and the PWV calculated by the system software using the measured surface distance between the two recording sites (SphygmoCor; AtCor Medical Systems Inc., Sydney, Australia). Right radial artery waveforms were recorded, and the pulse-wave analysis was used to generate a central (ascending aortic) waveform using the generalized transfer function and augmentation index. Central systolic, diastolic, and pulse pressures were derived. Luminal diameters during systole and diastole of the common carotid artery, ascending aorta, and abdominal aorta were measured using echocardiography, and the distension coefficients were calculated. The ascending aortic stiffness index was calculated as previously described.
The reproducibility of various vascular measurements in our laboratory was assessed in 10 healthy volunteers. The intraobserver and interobserver COVs were 2% and 7%, respectively, for CIMT, 4% and 17% for carotid to femoral PWV, 27% and 44% for common carotid distensibility, 26% and 29% for abdominal aorta distensibility, and 26% and 38% for FMD of the brachial artery.
Vascular-Ventricular Coupling
The ratio of arterial elastance (Ea) to LV end-systolic elastance (Ees) was calculated as an index of vascular-ventricular coupling using noninvasive methods as described and validated in adults. Ea was calculated as the ratio of end-systolic pressure to LV stroke volume. End-systolic pressure was estimated noninvasively as 0.9 × brachial systolic pressure. LV stroke volume was estimated by echocardiography as (velocity-time integral of the pulsed-wave Doppler trace of the LV outflow tract) × (aortic valve orifice cross-sectional area). Ees was estimated using a modified single-beat method as previously described, using brachial blood pressures, LV stroke volume, and estimated normalized Ees at arterial end-diastole calculated as the ratio of aortic pre-ejection time to total systolic time.
Biochemical Analysis
Blood tests were performed only in the obese group. Blood samples, obtained after overnight fasting, were analyzed for glucose, insulin, total cholesterol, high-density lipoprotein cholesterol, low-density lipoprotein cholesterol, and triglycerides according to the standard laboratory protocol of the Hospital for Sick Children. From the fasting glucose and insulin levels, the homeostasis model assessment was calculated as a measure of insulin sensitivity. Institutional normal values for biochemical parameters were available and are mentioned in the “Results” section when appropriate.
Data Analysis
Data are presented as mean ± SD. Independent t tests were used to assess the statistical significance of the differences between obese and control children for various parameters. Univariate linear regression analysis was used to identify associations between vascular or myocardial parameters (dependent variable) and BMI (independent variable), and multivariate regression analysis was used to adjust for a limited number of potential confounders. Confounders were chosen on the basis of previously published studies that showed associations with the investigated outcome variable and might potentially differ between obese and normal weight children (a priori selection). Pearson’s correlations and their statistical significance were calculated to investigate associations between myocardial and vascular parameters. All statistical analyses were performed using SAS version 9.2 (SAS Institute Inc., Cary NC).
Results
General Characteristics
The general characteristics of the study population are shown in Table 1 . The majority of the obese participants were severely obese (mean BMI percentile, 98.2 ± 2.0%). Systolic blood pressure and resting heart rate were higher in the obese group than in normal-weight controls. In the obese group, lipid abnormalities included high total cholesterol levels ( n = 3), high triglyceride levels ( n = 2) and both high cholesterol and high triglyceride levels ( n = 16) at the time of referral to the lipid clinic. Two obese participants were using cholesterol-lowering drugs (statins) at the time of vascular and myocardial assessment.
Controls | Obese subjects | ||||
---|---|---|---|---|---|
Variable | n | Value | n | Value | P |
Male gender | 27 | 22 (81%) | 21 | 17 (81%) | 1.00 |
Age (y) | 27 | 13.9 ± 2.3 | 21 | 14.2 ± 2.0 | .57 |
BMI (kg/m 2 ) | 27 | 18.9 ± 2.3 | 21 | 32.4 ± 4.9 | <.001 |
BMI percentile | 27 | 45.9 ± 22.7 | 21 | 98.2 ± 2.0 | <.001 |
Systolic blood pressure (mm Hg) | 27 | 108 ± 8 | 21 | 117 ± 14 | .01 |
Diastolic blood pressure (mm Hg) | 27 | 54 ± 6 | 21 | 57 ± 6 | .13 |
Resting heart rate (beats/min) | 27 | 63 ± 8 | 21 | 73 ± 15 | .01 |
Insulin (μIU/mL) | — | <15.8 ∗ | 15 | 24.3 ± 16.6 | — |
Glucose (mg/dL) | — | 59–110 ∗ | 21 | 92 ± 7 | — |
Triglyceride (mg/dL) | — | 35–115 ∗ | 21 | 166 ± 60 | — |
Total cholesterol (mg/dL) | — | 124–170 ∗ | 21 | 203 ± 52 | — |
High-density lipoprotein cholesterol (mg/dL) | — | 21–77 ∗ | 21 | 39 ± 8 | — |
Low-density lipoprotein cholesterol (mg/dL) | — | 59–137 ∗ | 21 | 131 ± 51 | — |
C-reactive protein (mg/L) | — | 0–8.0 ∗ | 21 | 2.88 ± 1.87 | — |
Homeostasis model assessment | — | — | 15 | 39.06 ± 27.98 | — |
Cardiac Geometry, Shortening Fraction, and Ejection Fraction
Absolute chamber dimensions, volumes, wall thickness, and LV mass were higher in obese children compared with age-matched and sex-matched normal controls ( Table 2 ). When geometric parameters were normalized for body size, only LV mass corrected for height was significantly different between both groups, while LV mass corrected for BSA was similar in obese and controls. Shortening fraction, ejection fraction, and systolic wall stress were not different between the obese group and normal controls.
Controls | Obese subjects | ||||
---|---|---|---|---|---|
Variable | n | Mean ± SD | n | Mean ± SD | P |
LADS (cm) | 27 | 2.9 ± 0.4 | 21 | 3.5 ± 0.5 | <.001 |
LA systolic area (cm 2 ) | 26 | 12.2+3.2 | 21 | 14.8+3.4 | .01 |
LA diastolic area (cm 2 ) | 26 | 6.6+2.2 | 21 | 7.9+1.9 | .05 |
RVEDD (cm) | 27 | 2.1 ± 0.39 | 21 | 2.4 ± 0.43 | .01 |
IVSEDD (cm) | 27 | 0.8 ± 0.1 | 21 | 0.9 ± 0.2 | .02 |
LVEDD (cm) | 27 | 4.6 ± 0.4 | 21 | 5.1 ± 0.5 | <.001 |
LVESD (cm) | 27 | 3.0 ± 0.4 | 21 | 3.2 ± 0.5 | .04 |
LVPWD (cm) | 27 | 0.7 ± 0.1 | 21 | 0.8 ± 0.2 | .01 |
LV length at diastole (cm) | 27 | 7.9 ± 0.9 | 21 | 8.1 ± 1.1 | .48 |
LVM (g) | 27 | 102.7 ± 31.1 | 21 | 149.6 ± 50.6 | .001 |
End-systolic systolic wall stress (dynes/cm 2 × 10 3 ) | 27 | 62.7 ± 13.8 | 21 | 59.0 ± 12.0 | .33 |
LADS-SDS | 27 | 0.04 ± 0.90 | 21 | 0.52 ± 1.33 | .17 |
RVEDD-SDS | 27 | 0.57 ± 1.06 | 21 | 0.60 ± 1.17 | .93 |
IVSEDD-SDS | 27 | 0.49 ± 1.06 | 21 | 0.60 ± 1.73 | .81 |
LVEDD-SDS | 27 | −0.08 ± 0.87 | 21 | −0.37 ± 1.44 | .42 |
LVPWD-SDS | 27 | −0.34 ± 1.10 | 21 | −0.21 ± 1.54 | .74 |
LVM adjusted for height (g/height 2.7 ) | 27 | 27.7 ± 5.3 | 21 | 36.0 ± 8.7 | .001 |
LVM adjusted for BSA (g/m 2 ) | 27 | 67.2 ± 13.3 | 21 | 72.9 ± 17.0 | .20 |
Shortening fraction ∗ (%) | 27 | 35 ± 5 | 21 | 37 ± 5 | .29 |
Ejection fraction † (%) | 25 | 60 ± 5 | 20 | 57 ± 7 | .08 |
∗ Calculated using M-mode echocardiography.
Pulsed Doppler and Pulsed-Wave Tissue Doppler
Early mitral and tricuspid inflow velocities (E) were not different between the obese and nonobese groups, but both mitral and tricuspid A-wave velocities were increased ( Table 3 ). Mitral inflow deceleration time, LV isovolumic relaxation time, and pulmonary venous systolic, diastolic, and atrial reversal velocities were not different between the study groups (data not shown). Systolic tissue Doppler velocities were borderline lower at the level of the mitral valve lateral annulus in the obese children but were not significantly different at the septal annulus and tricuspid valve lateral annulus. Septal A′ velocities were slightly higher in the obese group compared with the control group. No differences were observed in tissue Doppler E′ velocities at any of the three locations. E/E′ ratios were slightly higher in the obese group than in the controls at the septal and tricuspid lateral annular level but were similar at the mitral valve annular level.
Controls | Obese subjects | ||||
---|---|---|---|---|---|
Variable | n | Mean ± SD | n | Mean ± SD | P |
Pulsed Doppler velocities | |||||
Mitral valve E velocity (cm/sec) | 27 | 94 ± 12 | 21 | 98 ± 14 | .32 |
Mitral valve A velocity (cm/sec) | 27 | 37 ± 9 | 21 | 47 ± 14 | .008 |
Tricuspid valve E velocity (cm/sec) | 18 | 54 ± 13 | 21 | 58 ± 13 | .28 |
Tricuspid valve A velocity (cm/sec) | 18 | 26 ± 11 | 21 | 34 ± 11 | .04 |
Pulsed-wave tissue Doppler velocities | |||||
Mitral valve lateral E′ velocity (cm/sec) | 27 | 19.0 ± 2.4 | 21 | 19.1 ± 3.1 | .89 |
Mitral valve lateral A′ velocity (cm/sec) | 27 | 6.2 ± 2.0 | 21 | 6.9 ± 1.3 | .16 |
Mitral valve lateral S′ velocity (cm/sec) | 27 | 11.5 ± 2.2 | 21 | 10.3 ± 1.6 | .04 |
Mitral valve E/E′ ratio | 27 | 5.0 ± 0.7 | 21 | 5.2 ± 1.10 | .38 |
Septal lateral E′ velocity (cm/sec) | 27 | 15.5 ± 2.2 | 21 | 14.4 ± 2.0 | .07 |
Septal valve lateral A′ velocity (cm/sec) | 27 | 6.0 ± 1.4 | 21 | 7.0 ± 1.4 | .03 |
Septal valve lateral S′ velocity (cm/sec) | 27 | 8.6 ± 1.1 | 21 | 8.7 ± 0.9 | .83 |
Septal E/E′ ratio | 27 | 6.1 ± 1.1 | 21 | 6.9 ± 1.2 | .03 |
Tricuspid valve lateral E′ velocity (cm/sec) | 27 | 15.0 ± 2.0 | 19 | 13.9 ± 2.3 | .17 |
Tricuspid valve lateral A′ velocity (cm/sec) | 27 | 7.8 ± 1.9 | 19 | 8.8 ± 2.8 | .20 |
Tricuspid valve lateral S′ velocity (cm/sec) | 27 | 12.4 ± 1.2 | 20 | 12.4 ± 1.1 | .96 |
Tricuspid valve E/E′ ratio | 18 | 3.5 ± 0.9 | 19 | 4.3 ± 1.3 | .04 |
Myocardial Deformation Parameters
Intraobserver and interobserver COVs for CTD were 9% and 13% for radial systolic strain of the posterior wall, 18% and 9% for radial systolic strain rate of the posterior wall, and 18% and 23% for radial diastolic strain rate of the posterior wall. Intraobserver and interobserver COVs for STE were 6% and 13% for mean systolic longitudinal strain, 13% and 15% for mean longitudinal systolic strain rate, 13% and 10% for mean longitudinal diastolic strain rate, and 17% and 12% for mean systolic circumferential strain.
Assessment of global longitudinal strain and strain rate by STE and radial strain and strain rate of the posterior wall by CTD was possible in >95% of obese subjects and controls. End-systolic radial strain in the posterior wall of the left ventricle measured by CTD-derived deformation imaging was lower in obese subjects compared with controls, while peak systolic and diastolic strain rate values were similar between both groups ( Figure 1 ). Mean STE-derived longitudinal systolic strain, systolic strain rate, and diastolic strain rate values of the left ventricle were lower in the obese group ( Figure 2 ). Systolic strain values were lower in five of the six segments of the left ventricle, with the exception of the basal lateral segment (data not shown). Mean STE-derived circumferential strain was −17 ± 3% for obese subjects and −18 ± 2% for controls ( P = .12).


Vascular Measurements
The results of the vascular measurements are summarized in Table 4 . In obese patients, CIMT was higher, PWV was faster, central systolic pressure and pulse pressure were higher, augmentation index was higher, and carotid and ascending aortic distension coefficients were lower compared with controls. No differences were observed in flow-mediated vasodilatation of the brachial artery, abdominal aortic distension coefficient, and ascending aorta stiffness index between the groups.
Controls | Obese subjects | ||||
---|---|---|---|---|---|
Variable | n | Mean ± SD | n | Mean ± SD | P |
CIMT (mm) | 27 | 0.42 ± 0.05 | 20 | 0.48 ± 0.08 | .01 |
FMD (%) | 27 | 8.3 ± 4.0 | 20 | 7.2 ± 3.4 | .33 |
PWV (m/sec) | 27 | 4.7 ± 1.0 | 21 | 5.9 ± 1.1 | <.001 |
Central systolic blood pressure (mm Hg) | 27 | 86 ± 7 | 21 | 96 ± 12 | .003 |
Central diastolic blood pressure (mm Hg) | 27 | 55 ± 6 | 21 | 58 ± 7 | .11 |
Central pulse pressure (mm Hg) | 27 | 31 ± 5 | 21 | 37 ± 9 | .009 |
Augmentation index (%) | 27 | −11 ± 13 | 21 | −1 ± 12 | .009 |
Carotid distension coefficient (mm Hg −1 ) | 27 | 0.5 ± 0.1 | 20 | 0.4 ± 0.1 | .01 |
Ascending aortic distension coefficient (mm Hg −1 ) | 27 | 0.4 ± 0.1 | 18 | 0.3 ± 0.1 | .02 |
Abdominal aortic distension coefficient (mm Hg −1 ) | 23 | 0.5 ± 0.1 | 14 | 0.4 ± 0.2 | .13 |
Ascending aortic stiffness index | 27 | 3.9 ± 1.5 | 18 | 4.7 ± 1.7 | .12 |
Myocardial and Vascular Parameters: Association with BMI
The associations between BMI and myocardial deformation parameters using univariate and multivariate regression analysis are shown in Table 5 and Supplemental Table 1 . The results for the obese and control children were grouped. Radial strain by CTD was significantly associated with BMI, but the association was no longer statistically significant after adjusting for LV end-diastolic dimensions, while systolic blood pressure and LV wall stress did not have a major impact ( Supplemental Table 1 ). BMI was also associated with longitudinal deformation. Adjustment for systolic blood pressure, heart rate, LV wall stress, LV mass, and LV dimensions did not have a major impact on the association between BMI and longitudinal deformation parameters. For the obese children, data on serum lipid profiles, glucose, insulin levels, and homeostasis model assessment were further explored as additional explanatory factors. Adjustment for these measurements did not have a major impact on the association between BMI and myocardial deformation parameters (data not shown).
Univariate analysis | Multivariate analysis ∗ | |||||
---|---|---|---|---|---|---|
Variable | Regression estimate | SE | Adjusted R 2 | Regression estimate | SE | Adjusted R 2 |
Radial systolic strain (CTD) | −0.80 † | 0.22 | 0.22 | −0.67 § | 0.37 | 0.30 |
Longitudinal systolic strain (STE) | 0.228 † | 0.04 | 0.40 | 0.187 ‡ | 0.07 | 0.37 |
Longitudinal systolic strain rate (STE) | 0.016 † | 0.003 | 0.29 | 0.014 ‡ | 0.006 | 0.23 |
Longitudinal diastolic strain rate (STE) | −0.054 † | 0.007 | 0.58 | −0.056 † | 0.012 | 0.58 |
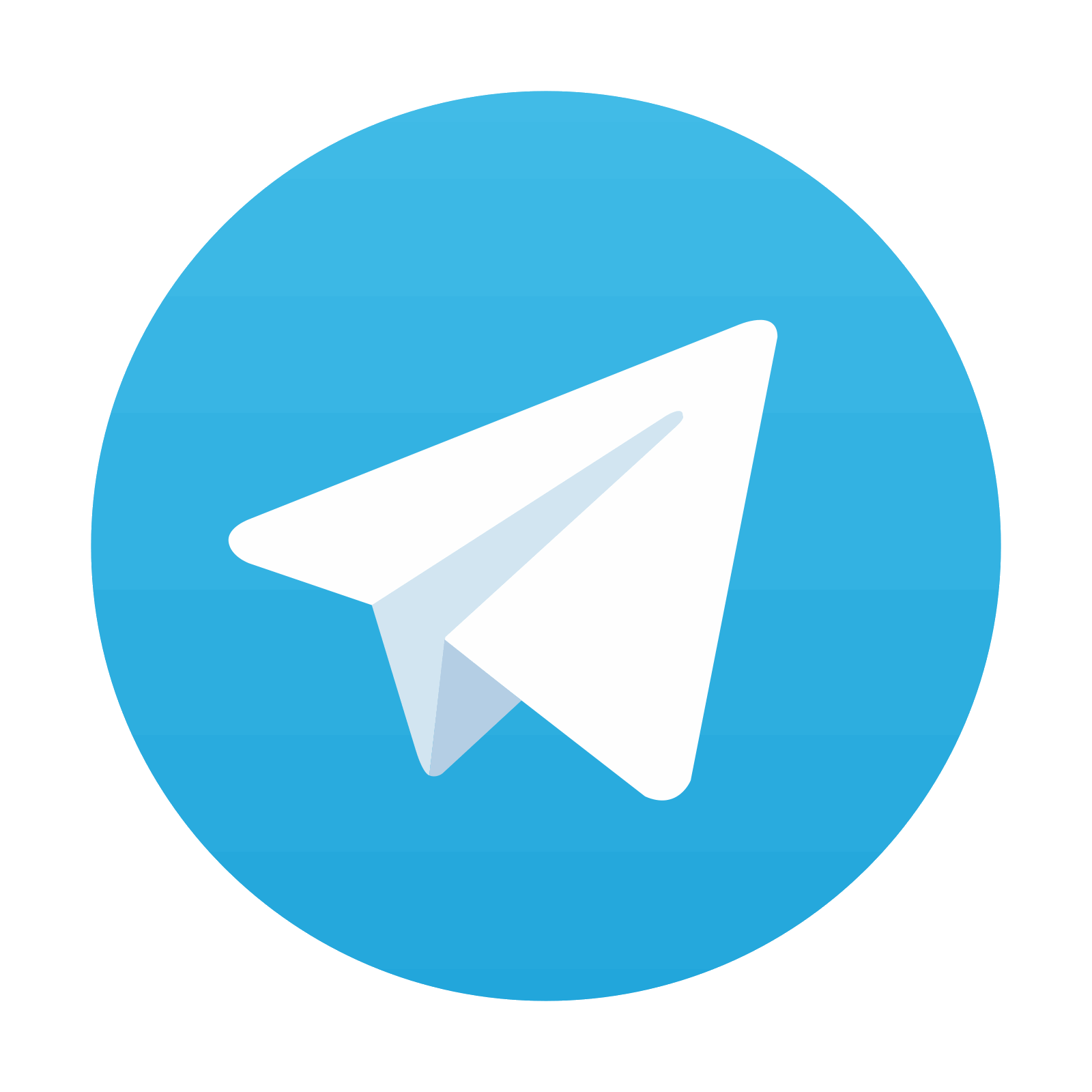
Stay updated, free articles. Join our Telegram channel
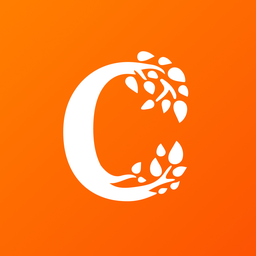
Full access? Get Clinical Tree
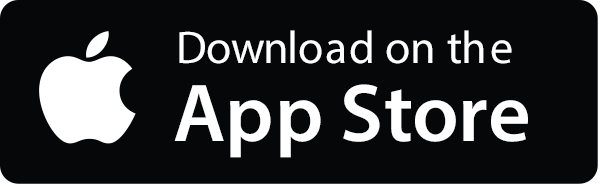
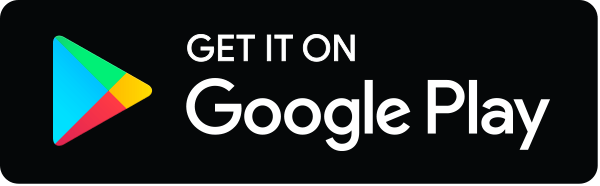
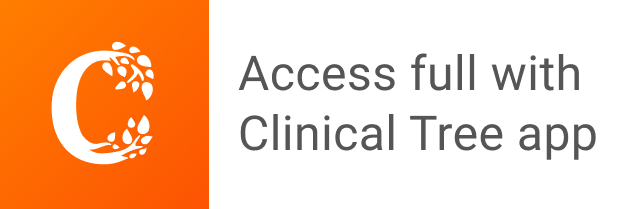