Chapter 8 Intensive care for the critically ill adult
INTRODUCTION
There remains a perception by some medical disciplines that physiotherapy patient management in the intensive care unit (ICU) is focused solely on the maintenance and improvement of a patient’s cardiopulmonary status. However, the role of the physiotherapist also includes maintenance of musculoskeletal function, optimization of neurological status, and is extending to areas such as extubation/decannulation, ventilator weaning, troubleshooting mechanical ventilation problems and therapeutic fibre optic bronchoscopy (Jones 2001, Norrenberg & Vincent 2000) and involvement in ‘patient at risk’ and medical emergency teams.
Advances in technology and pharmacology have contributed to the increasing survival of patients from critical illness. Dowdy et al (2005) suggest that ICU survivors have a significantly lower quality of life, both before and after ICU stay, compared with the general population. The impact of post-ICU discharge rehabilitation on a patient’s functional capacity and quality of life is as yet unclear.
Physiotherapists may have a role in enhancing functional reserve before patients are admitted to ICU (prehabilitation). This should enable patients to better withstand the stress of ICU procedures and inactivity (Topp et al 2002). It follows that the place of physiotherapy in the management of patients in the ICU comprises a three-segment continuum of care:
Irrespective of the evolving direction of the place of physiotherapy in ICU, optimization of the cardiorespi ratory status of the patient remains a central objective. It is therefore essential that the physiotherapist has:

MONITORING AND MECHANICAL SUPPORT
Mechanical ventilation
Mechanical ventilation is essential to maintain life for some patients and is often used for patients with respiratory failure (Box 8.1).
Box 8.1 Types of respiratory failure
Type 1: | |
Type 2: | |
Hypoxaemia = PaO2 <60 mmHg with FiO2 >0.5 | |
Abbreviations: CO2, carbon dioxide; PaO2, partial pressure of oxygen in arterial blood; FiO2, fractional inspired oxygen concentration |
Hypoxaemia = PaO2 <60 mmHg with FiO2 >0.5
Full ventilatory support should maintain or improve alveolar ventilation (Pilbeam 1992) and reduce the work of breathing. Work of breathing is an important consideration during the provision of mechanical support and can be reduced with various types of inspiratory support. This may be achieved by supporting respiration in various modes such as control, assist-control, synchronized intermittent mandatory ventilation (SIMV) with or without pressure support and bilevel positive airway pressure ventilation (BiPAP). The ventilator pressure waveforms of some common modes of ventilatory support are illustrated in Figure 8.1.
The following descriptions are summarized from the review by Weavind & Wenker 2000.
Airway pressure release ventilation (APRV)
This mode of ventilation maintains continuous positive pressure in the airway (CPAP) with intermittent release of the pressure (essentially an inverse ratio form of bilevel ventilation). The duration of release is rather short, and similar to PC–IRV, and results in an inverse I : E ratio mode of ventilation. Patients who are able to breathe spontaneously with a relatively low work of breathing can utilize APRV, thereby minimizing barotrauma and circulatory compromise. This mode of ventilation may not be suitable for patients with asthma or severe COPD because they tend to find difficulty in emptying their lungs during the short release.
Biphasic positive airway pressure (BiPAP) and bilevel ventilation
BiPAP is pressure-controlled ventilation with two levels of continuous positive airway pressure (CPAP) (Phigh and Plow), with a set breathing rate. The I : E ratio can be adjusted. Bilevel ventilation is a combination of APRV and BiPAP. The mandatory breaths are pressure-controlled and the spontaneous breaths can be pressure supported (often only at Plow). Thus, bilevel ventilation can be used as pressure-controlled ventilation initially in sedated or paralysed patients, then weaned to CPAP and PS (to allow spontaneous breathing) and then to CPAP. Figure 8.2 shows the biphasic waveform on a monitor screen.
High-frequency ventilation
High-frequency ventilation can be jet ventilation or oscillation. In jet ventilation, a high pressure (30–300 kPa) of air with supplementary oxygen is delivered to the airway via a small-bore catheter at frequencies between 60 and 300 Hz. Expiration is passive. This form of ventilation is mainly used during surgery when the airway is disrupted or placement of a tracheal tube is not possible. High-frequency oscillatory ventilation (HFOV) (Fig. 8.3) is oscillation of a continuous distending pressure at rates of 100–1000/min with active inspiration and expiration. The advantage of high-frequency oscillatory ventilation is a stable continuous positive airway pressure, with control of ventilation at high breath rates and small tidal volumes (50–100 ml). The patient needs to be very heavily sedated and/or paralysed to minimize or prevent spontaneous respiration, often resulting in the cough reflex being abated. Humidification is often inadequate with this form of ventilatory support.
Other forms of ventilation and adjuncts
Inhaled nitric oxide can be used as a selective pulmonary vasodilator. It has been used for many decades for the management of severe arterial hypoxaemia and pulmonary hypertension in both adults and children. Recent guidelines (Germann et al 2005) recommend inhaled nitric oxide as useful rescue therapy for the management of severe pulmonary arterial hypertension and severe refractory arterial hypoxaemia, but do not confer any survival benefits in adults.
‘Protective’ and ‘open lung’ ventilation are currently used for such conditions as acute lung injury and acute respiratory distress syndrome. This consists of using low tidal volumes (6–8 ml/kg), allowing the partial pressure of carbon dioxide in arterial blood (PaCO2) to rise and ensuring the alveoli remain inflated with optimal positive end-expiratory pressure (PEEP) (Levitt & Matthay 2006). There is Level 1 evidence (Petrie et al 1995) for low tidal volumes causing a decrease in mortality (Petrucci & Iacovelli 2004).
Monitoring of the body systems
Monitoring of patients on mechanical ventilation
Monitoring respiratory muscle function.
Respiratory muscle weakness is often associated with prolonged mechanical ventilation and the cause of failure or delay in weaning. Diaphragmatic strength can be determined non-invasively in mechanically ventilated patients and can be assessed from the twitch gastric, twitch oesophageal and twitch transdiaphragmatic pressures in response to phrenic nerve stimulation (Mills et al 2001). Maximal static inspiratory and expiratory efforts, electromyography of respiratory muscles, respiratory rate, carbon dioxide (CO2) level, pressure–time product, vital capacity and maximum voluntary ventilation are all variables which reflect respiratory muscle function. Respiratory rate and tidal volume presently remain the most convenient and frequently used indices of respiratory muscle function (e.g. respiratory rate/tidal volume provides a rapid shallow breathing index) (Spicer et al 1997). These measures may be used to assess the readiness of the patient to breathe spontaneously and to be weaned from ventilatory support.
Lung mechanics.
The change in lung volume and pressure are measured at end-inspiration (compliance) and end-expiration (auto-peep); this is when airflow ‘momentarily’ ceases during the ‘normal’ respiratory cycle. Most mechanical ventilators in ICU display/calculate dynamic lung compliance, auto-peep as well as airway resistance.
Assessment of work of breathing.
To optimize mechanical ventilation, the mechanical ventilator must promptly respond to the patient’s demand during inspiration and allow an unimpeded expiration. Mechanical ventilation practices vary internationally and hence patient management varies, depending on institutionally driven, airway and ventilator strategies (Esteban et al 2000).
Monitoring of the respiratory system
Monitoring oxygenation
Blood gases. Arterial blood gases (partial pressure of oxygen and carbon dioxide, and pH) provide essential information about a patient’s metabolic as well as respiratory status. Continuous measurement of arterial blood gases is possible but this technique is not widely practised because of cost, calibration drift and clot formation (Shapiro et al 1993, Zimmerman & Dellinger 1993). Interpretation of arterial blood gases is discussed in Chapter 3.
Transcutaneous gas measurement. This method of measurement is based on the principle that blood flow and oxygen exchange are dependent on skin temperature (Baumburger & Goodfriend 1951). Transcutaneous electrodes include a heating element to maximize local blood flow. When the skin is heated the capillary blood becomes ‘arterialized’ and the arterialized gases diffuse through the skin to the electrodes (Whitehead et al 1980). More recently, a new sensor (TOSCA monitor) for combined continuous transcutaneous monitoring of arterial oxygen saturation and carbon dioxide tension has been shown to be an accurate and valuable tool for respiratory monitoring (Bernet-Buettiker et al 2005, Senn et al 2005). At present this is used more commonly in neonates.
Oximetry. Non-invasive assessment of oxygen saturation by a pulse oximeter (SpO2) was introduced clinically in 1975 (Kendrick 2001). Pulse oximetry is now an expected monitoring component for assessment of hypoxaemia. The oxygen saturation of arterial blood [with partial pressure of oxygen (PO2) of 100 mmHg or 12–13 kPa] is about 97.5% and that of mixed venous blood (with PO2 of 40 mmHg or 5–5.5 kPa) is about 75% (West 2005). The absorption of light energy by blood varies, depending on the wavelength. Red (660 nm) and infrared (940 nm) light result in the greatest separation between deoxyhaemoglobin and oxyhaemoglobin absorption spectra. Pulse oximetry uses light-emitting diodes set at these wavelengths, and as the emitted light passes through the finger (or earlobe), the light energy is variably absorbed by the arterial and venous blood. The absorption ratio of the red/infrared light by the blood is proportional to the amount of desaturated haemoglobin, and from these data the pulse oximeter calculates and displays the SpO2 (Kendrick 2001).
The accuracy of commercial pulse oximeters is about ±2% within the clinical oxygen saturation range of 70–100% (Jensen et al 1998). Pulse oximeter readings may be inaccurate in patients with severe or rapid desaturation, hypotension, hypothermia and low perfusion states. A further limitation of oximetry is that it provides information only on oxygen status, and not on ventilation (PaCO2).
Monitoring carbon dioxide (capnography).
The concentration of carbon dioxide expired during different phases of the respiratory cycle provides information on the effectiveness of alveolar ventilation (Fig. 8.4), not only the end-tidal carbon dioxide value but also tube position and breathing circuit integrity.
Detailed ‘indications’ for capnography can be found in the American Association for Respiratory Care (AARC) Clinical Practice Guidelines on Capnography/Capnometry during Mechanical Ventilation (AARC 1995).
Monitoring of the cardiovascular system
Non-invasive monitoring
Blood pressure. The use of automated non-invasive blood pressure (NIBP) devices is common. The mean arterial pressure (MAP) most closely approximates capillary perfusion pressure and is thus a useful measurement. NIBP devices can give inaccurate results in patients with arrhythmias, cardiac valvular lesions, where there is improper cuff application and when the blood pressure is low (Box 8.2).
Box 8.2 Normal values for blood pressure (BP)
Normal value of systolic/diastolic pressure
Normal value of mean arterial pressure
Electrocardiogram. The electrocardiogram provides information on the rate and rhythm of the heart; it also assists in the diagnosis, and identification of the possible site of myocardial infarction. The pathological feature of myocardial infarction is necrosis of myocardial muscle. Absolute evidence of myocardial necrosis is the pathological Q wave. Q waves, ST elevation and T wave inversion are all associated with transmural infarction (involvement of whole thickness of the myocardium). For sub-endocardial infarction (involvement of the inner layer of the myocardium, adjacent to the endocardium), Q waves do not appear and changes are confined to ST segments and T waves. Thus subendocardial infarction may be difficult to differentiate from ischaemia of the myocardium. Interpretation of the electrocardiogram is discussed in Chapter 3.
Thoracic electrical bioimpedance and impedance cardiography. Thoracic electrical bioimpedance (TEB) relies on measurement of bidirectional blood flow within the aorta by a laser Doppler velocimeter and an impedance measurement unit which determines the cross-sectional area of the vessel. TEB allows the clinician to view beat-to-beat cardiac output. (Newman and Callister 1999, Tjin et al 2001) and provides information on haemodynamic indices such as systolic time interval, left cardiac work index and end diastolic index (Belott 1999, Weiss et al 1997).
Non-invasive measurement of stroke volume and cardiac output by impedance cardiography (ICG) has been shown to be accurate and significantly correlated to conventional thermodilution method (see Thermodilution cardiac output, below) (Scherhag et al 2005). ICG measures synchronized pulse changes in TEB via simple surface electrodes together with a conventional electrocardiogram.
Partial CO2rebreathing cardiac output and NICO. Rather than applying the oxygen Fick method for cardiac output monitoring, the non-invasive CO2 Fick methods for estimation of cardiac output are receiving increased clinical interest. Adopting the CO2 version of the Fick equation has the advantage that CO2 elimination is easier to measure accurately compared with oxygen uptake (Jaffe 1999). The differential Fick partial rebreathing method computes a cardiac output value based on the changes in carbon dioxide elimination and end tidal CO2 in response to a change in ventilation. The non-invasive cardiac output (NICO system) is the first commercially available cardiac output system making use of the principle of partial rebreathing of CO2 (Jaffe 1999).
Echocardiography and Doppler. This uses ultrasound to examine the performance of the heart and great vessels. Information from echocardio-graphy can be presented one- (M-mode), two- or three-dimensionally. M-mode is often used in conjunction with a two-dimensional echo to provide a clear illustration of the structures being investigated (Young & Sanderson 1997).
Invasive monitoring
Arterial pressure. Intra-arterial measurement should normally be considered accurate, but the systolic pressure may be overestimated due to systolic ‘overshoot’ (a property of the fluid-pressure transducer monitoring system). The ‘area’ under the arterial tracing can provide a rough estimate of the cardiac output (Gomersall & Oh 1997).
Central venous pressure. Central venous pressure (CVP) reflects right ventricular filling and is usually monitored by a catheter inserted via the internal jugular or subclavian vein, and less frequently via the femoral vein. A quick way to confirm correct placement of the catheter is observed pressure change with respiration (Gomersall & Oh 1997). As the right ventricular preload is determined by the volume and not the pressure, the absolute value of CVP is less meaningful. A high CVP value, however, may be associated with conditions that cause a rise in the right atrial pressure (for example right heart failure, reduced right ventricular diastolic compliance, hypervolaemia and pulmonary hypertension) (Gray et al 2002), whereas a low CVP value may suggest hypovolaemia. Changes in CVP may provide useful guidance for fluid management in patients: for example, a minimal rise of CVP despite fluid loading may suggest the loading volume is insufficient; but a rise of CVP of more than 9.5 cmH2O (7 mmHg) may indicate maximal loading. A raised CVP in response to fluid loading is expected to return to its original value within 10 minutes – an indication that the risk of pulmonary oedema is only moderate (Gomersall & Oh 1997) (Box 8.3).
Thermodilution cardiac output. Non-invasive monitoring of cardiac output has been discussed above. Traditional measurement of cardiac output by the thermodilution method requires the use of a pulmonary artery catheter. Cardiac output can be computed from the decrease in blood temperature in the pulmonary artery after injection of a known volume of cold saline into the right atrium. Computation of cardiac output is based on the principle that the decrease in blood temperature is inversely proportional to the extent of dilution of the cold saline (i.e. the higher the cardiac output the less the change in temperature between injection and measurement points).
Continuous thermodilution is possible by monitors that use infusion of heat from a filament in the right atrium rather than injection of cold saline. The monitor displays cardiac output averaged over the previous 3 to 6 minutes (Boldt et al 1994, Yelderman et al 1992).
The most commonly used method of continuous cardiac output monitoring is by PiCCO® technology. This technology is less invasive. It requires the insertion of a thermodilution catheter in the femoral or axillary artery and a central venous catheter. (The use of a right heart catheter is not necessary.) This technology is based on the transpulmonary thermodilution technique and arterial pulse contour analysis and provides specific and quantitative parameters including arterial blood pressure, heart rate, cardiac output, global end-diastolic volume [an indicator of cardiac volume, the normal range of global end-diastolic volume index (GEDVI) is 680–800 ml/m2], intrathoracic blood volume [an indicator of thoracic blood volume, the intrathoracic blood volume index (ITBVI) is 850–1000 ml/m2], extravascular lung water [an indicator of pulmonary oedema, extravascular lung water index (EVLWI) is 3.0–7.0 ml/kg], cardiac function index, global ejection fraction, stroke volume, stroke volume variation SVV (an indicator of the potential for a response to intravascular filling, SVV should be less than 10%), pulse pressure variation (also an indicator of the potential for a positive response to intravascular filling) and systemic vascular resistance (an indicator of left ventricular afterload). (Pulsion Medical Systems) (Fig 8.5).
Monitoring of the neurological system
Level of consciousness
The Glasgow Coma Scale (Chapter 1) is the most common way to objectively index the level of consciousness. Pupil size and level of reactivity to light provides an index of neurological integrity (pupils equal and reactive to light (PERL)). A fixed dilated unilateral pupil indicates pressure on the oculomotor nerve and urgent investigation is necessary. Physiotherapy intervention should be delayed. Fixed dilated pupils indicate severe neurological impairment, which may be made worse by hypoxia or biochemical abnormalities and are often a sign of brainstem death.
Cranial computed tomography scan.
Computed tomography (CT) of the head provides information about the brain and skull. A plain skull radiograph may identify fractures of the skull and CT with contrast is used for investigation of intracranial space-occupying lesions (haemorrhage, tumour or abscess). Cranial CT permits visualization of the following (Kumar 1997):
Magnetic resonance imaging (MRI) is now more commonly used when scanning neurological patients.
Intracranial pressure.
Intracranial pressure (ICP) is measured by insertion of a catheter through the skull into the lateral ventricle or by means of an extradural or subarachnoid bolt (Turner 2002). ICP is often monitored in patients with head injuries, post-brain surgery and for patients with intracranial and subarachnoid haemorrhage or cerebral oedema. The intraventricular catheter has the advantage of allowing drainage of cerebrospinal fluid when the intracranial pressure is high but because it penetrates the dura, there is a greater accompanying risk of intracranial infection. Any change in the intracranial pressure is dependent upon the relative amounts of blood, brain and CSF within the adult skull. ICP allows determination of global cerebral perfusion pressure (CPP), which relates closely to cerebral blood flow (CBF) (Box 8.4).
Box 8.4 Calculation of cerebral perfusion pressure (CPP)
CPP = cerebral perfusion pressure; MAP = mean arterial pressure; ICP = intracranial pressure
Raised PCO2 results in an increase in cerebral blood flow, which will cause a rise in ICP and a lowering of CPP. Hyperventilation may lower the PCO2, thus reducing cerebral vasodilatation and CBF, thereby lowering the ICP. Normal ICP is 10–15 mmHg, but baseline levels are often higher in neurosurgical patients. In order to provide adequate perfusion to the brain, it is generally recommended that CPP should be maintained at a level greater than 60 mmHg (Huang et al 2006).
Jugular bulb oxygen saturation.
Jugular bulb oxygen saturation (SjO2) reflects the adequacy of global cerebral oxygen delivery, although monitoring of SjO2 is now rarely used in neurosurgical ICU. Monitoring of SjO2 is based on the principle that cerebral arterial and mixed venous oxygen difference (A–VDO2) is directly proportional to cerebral metabolic rate (CMRO2) but inversely proportional to cerebral blood flow (CBF). The normal values of SjO2 are between 55–71%. Less than 55% indicates an increase in cerebral oxygen extraction, often due to hypotension or systemic hypoxia. Over 70% indicates hyperaemia. The results have to be interpreted along with other information such as ICP (White & Baker 2002).
Measurement and monitoring of cerebral blood flow
Transcranial Doppler. Transcranial Doppler (TCD) is a non-invasive diagnostic tool that uses sound waves to measure the velocity of blood flow in the basal cranial arteries (Miller 2005). Blood flow velocity, however, is variable and dependent on the diameter of the cerebral arteries. Thus a dimensionless variable, the pulsatility index (PI) is used which is derived from the difference between systolic and diastolic flow velocities divided by the mean velocity. PI is a reflection of the cerebrovascular resistance and a high PI is associated with a low cerebral perfusion pressure (CPP) (Lindegaard 1992). A high correlation between PI and ICP (Voulgaris et al 2005) and CPP (Bellner et al 2004) has been reported. PI has a high predictive value for detecting a CPP of less than 70 mmHg (Voulgaris et al 2005).
Bispectral index (electroencephalographic analysis). The bispectral index (BIS) is a parameter that was developed to measure a patient’s level of awareness during sedation (Haug et al 2004) and to determine the probability of recovery of consciousness in patients in coma (Fabregas et al 2004). BIS is derived from an electroencephalogramic parameter, which includes time and frequency domains and higher-order spectral information. With electrode sensors placed on the forehead of the healthiest brain hemisphere, identified by computed tomography scan, a BIS recording can be quantified on a scale from 0 to 10. BIS correlates with clinical signs of hypnosis (Billard et al 1997, Rosow and Manberg 2001) and is reported to be predictive of traumatic brain injury and neurological outcome at discharge (Haug et al 2004).
PROBLEM IDENTIFICATION AND PHYSIOTHERAPEUTIC INTERVENTIONS IN ICU
Broad problems
Chapter 6 has comprehensively detailed potential problems that may occur in the respiratory patient, mechanisms, medical and physiotherapy management. Apart from the presenting problem, for example, severe pneumonia or multiple trauma, patient’s previous comorbidities, immobility, problems associated with intubation, ventilation and impaired nutrition should be considered. Problems particularly relevant to critically ill patients include:
Decreased lung volumes, compliance and gas exchange
Intubation, mechanical ventilation and the accompanying sedation can result in a number of adverse effects on the respiratory and cardiovascular system. Ventilation/perfusion mismatching may occur due to preferential ventilation of the non-dependent areas (increased dead space) of the lung while the poorly ventilated dependent areas still receive preferential perfusion (increased shunt) especially in the supine position. The monotonous pattern of positive pressure ventilation without spontaneous respiration may impair gas exchange (Hedenstierna et al 1985) and the absence of sighs during mechanical ventilation will lead to decreased surfactant release, decreased lung compliance and progressive pulmonary atelectasis (Antonaglia et al 2006). Decreased functional residual capacity (FRC) also occurs because of cephalad displacement of the diaphragm and loss of lung volumes, both of which occur predominantly in the dependent zones. Alveoli may develop different levels of resistance, those with high resistance taking a longer time to inflate. The different mechanical properties of alveoli may be interpreted as having varying time constants (the product of alveolar compliance × resistance). A long time constant indicates an alveolus that opens slowly during tidal inflation.
Decreased mucociliary/secretion clearance
Intubation and mechanical ventilation can inhibit the normal mucociliary clearance and be associated with secretion retention and pneumonia (Konrad et al 1994). A patient intubated and ventilated for longer than 48 hours has been shown to be heavily colonized with anaerobic bacteria (Agvald-Ohman et al 2003). The colonization of bacteria may be partly due to suction-induced lesions of mucous membranes. These bacteria are then capable of synthesizing and releasing factors capable of further impairing ciliary mobility and causing a loss in epithelial integrity. It is important that the physiotherapist understands the mechanisms of impaired mucociliary clearance in the intubated ventilated patient and appreciates which methods of intervention are effective.
Weakness of respiratory and peripheral muscles
Mechanical ventilation for as little as 48 hours has been demonstrated to decrease diaphragm strength (Sassoon et al 2002) and endurance of respiratory muscles (Chang et al 2005).
A combination of the catabolic effects of the major illness, stress response, hospital-acquired infections and certain pharmacological agents can result in the loss of large amounts of muscle mass attributed to a proteolytic or protein degradation process or specific critical care weakness syndromes (Latronico et al 2005). General immobility also results in clinically significant bone demineralization and general impairment of orthostatic reflexes. This can result in increased time on mechanical ventilation, longer hospital stay and decreased quality of life on discharge.
Increased work of breathing
The primary reasons for mechanical ventilation are to decrease the work of breathing and optimize gas exchange. Mechanical ventilation can be applied to patients who are or are not making spontaneous respiratory efforts (Georgopoulos et al 2006). The patient’s respiratory system may either be passively ventilated (mandatory modes) or the patient may interact with the ventilator and trigger machine- supported breaths (in synchronized modes with set tidal volume or set pressure), or the patient may spontaneously breathe throughout the respiratory cycle interspersed with positive pressure breaths (bilevel ventilation).
There are many means of assessing the lung/thorax mechanics, work of breathing and metabolic cost of breathing in an intubated and mechanically ventilated patient (Table 8.1).
Table 8.1 Measures of work of breathing, lung thorax/mechanics and metabolic consumption
P0.1 = effort in first 100 msec in inspiration, NIF = negative inspiratory force, MIP = maximal inspiratory pressure, PEEP = positive end-expiratory pressure, f/Vt = rapid shallow breathing index, bpm = breaths per minute
Interventions
Positive pressure
Hyperinflation techniques.
The aims of hyperinflation techniques are to:

Manual hyperinflation (MHI) is a technique whereby the patient is disconnected from the ventilator and given an altered breathing pattern via a valve circuit and reservoir bag (Fig. 8.6). To reduce the risk of barotrauma, it is recommended that a pressure manometer be incorporated into the circuit so that the peak airway pressure can be monitored during the MHI procedure.
Various types of circuits are utilized. Expiratory flow rate and volume produced by the more pliable Mapleson and Magill circuits and the less pliable Air Viva and Laerdal circuits have been compared, both in laboratory and clinical studies (Jones et al 1991, 1992a, 1992b, Maxwell & Ellis 2003, McCarren & Chow 1998, Rusterholz & Ellis 1998). The Mapleson and Magill circuits provide greater tidal volume and faster expiratory flow. When a pressure manometer is not in use, the latter circuits may be safer.
Ventilator hyperinflation (VHI) has been used as an alternative to manual hyperinflation in ventilated patients. The method consists of leaving the patient attached to the ventilator and altering either the volume, pressure or flow/time characteristics of the breath delivered. This method of ventilation has been shown to result in similar levels of secretions removed and improvements in compliance as manual hyperinflation (Berney & Denehy 2002, Savian et al 2005).
Evidence for hyperinflation techniques
Effect on decreased volumes, compliance and oxygenation. As discussed, the critically ill patient may have low lung volumes and decreased lung compliance due to a number of factors. In order to reverse the process of decreased lung compliance, the inspiratory pressure must exceed a critical opening level to expand collapsed alveoli. A pressure of 40 cmH2O has been stated to be a minimum (Rothen et al 1999); however, other studies have resolved atelectasis using lower pressures (Maa et al 2005).
MHI has been demonstrated to result in reversal of atelectasis (Stiller et al 1990), improvement in tidal volumes, improvement in chest radiograph scores (Maa et al 2005), improvements in static and dynamic compliance (Hodgson et al 2000, Jones et al 1992b, Paratz et al 2002, Patman et al 2000) and increased yield of secretions (Choi & Jones 2005, Hodgson et al 2000, Jones 2002).
Effect on mucociliary clearance. The fast release technique during the expiration phase of MHI encourages movement of the secretions in a cephalad direction. Patients on mechanical ventilation are normally sedated, with a consequence of reduced ability to cough. The movement of airway secretions in these patients is impaired and may be improved by an increased expiratory to inspiratory flow ratio (the ‘two-phase gas liquid transport’), which is proposed to occur during MHI or VHI (Savian et al 2005).
Laboratory and clinical studies have demonstrated that manual hyperinflation compared with a control manoeuvre results in higher peak expiratory flows, improved lung/thorax compliance and increased removal of secretions (Jones 2002). It appears that a critical expiratory flow must be reached during manual hyperinflation in order for sputum movement to occur and this is linked to the method of delivery of manual hyperinflation, including the type of circuit employed (Maxwell & Ellis 2003, Savian et al 2005); tidal volume; and rapid release of the valve and bag. Research on MHI to date has not established a link between the flow rates generated and the volume of secretions removed.
Inclusion of a positive end-expiratory pressure (PEEP) valve in the manual hyperinflation circuit has been shown to decrease expiratory flow. When the PEEP exceeds 10 cmH2O, MHI may be ineffective as a secretion clearance technique (Savian et al 2005).
Laboratory evidence has suggested that MHI may not be effective in mobilization of thin (low viscosity) secretions and an alternative technique such as gravity-assisted drainage may be more effective (Jones 2002).
A further method of hyperinflation that intensivists and some physiotherapists utilize are ‘recruitment manoeuvres’ (Mols et al 2006). Lung-protective strategies using low tidal volume ventilation are beneficial and improve survival in patients with acute respiratory distress syndrome. However, the low tidal volumes can cause tidal alveolar de-recruitment and atelectasis. A recruitment manoeuvre is a sustained increase in airway pressure with the goal to recruit atelectatic lung tissue. A number of methods are used, including increases in positive end-expiratory pressure (PEEP), sustained increased inspiratory pressure (e.g. 40 cmH2O PEEP for 40 seconds duration), or sigh breaths. Using these techniques, short-term increases in oxygenation and reversal of atelectasis have been reported (Barbas et al 2005).
Precautions for hyperinflation techniques



High PEEP, nitric oxide, heat and moisture exchanger (HME) and hypoxic pulmonary vasoconstriction (HPV). If the patient is receiving high PEEP (>10 cmH2O) and/or nitric oxide as a ventilatory adjunct, it is advisable not to disconnect the ventilator circuit for manual hyperinflation techniques. Interruption to high levels of PEEP can cause de-recruitment and atelectrauma, especially in conditions such as ARDS (Mols et al 2006). Interruption of nitric oxide can cause sudden increases in pulmonary artery pressure and severe strain on the right side of the heart as well as potential severe hypoxaemia. Ventilator hyperinflation may be an alternative in these situations, but may dilute the amount of nitric oxide the patient is receiving, unless the patient is on an automated nitric oxide delivery device.
Non-invasive ventilation (NIV)
Biphasic positive airway pressure (BiPAP), continuous positive airway pressure (CPAP) and intermittent positive pressure breathing (IPPB) have been covered earlier and in Chapters 5 & 10. These modes of ventilation are of particular use in the critically ill patient in attempting to prevent intubation in respiratory failure or in weaning and extubation. Patients with chronic obstructive airways disease, chronic heart failure, obesity and renal failure are often at risk of needing reintubation and ventilation following extubation. These patients may benefit from some form of NIV post-extubation.
Manual techniques
Percussion and vibration.
Although manual techniques such as percussion of the chest wall and vibration during the expiratory phase are commonly used in intensive care patients, often in conjunction with hyperinflation techniques and positioning, individual studies of their effectiveness in this setting are currently lacking. Vibration has been shown to increase expiratory flow rate (MacLean et al 1989), but there are no clinical studies that demonstrate whether this increases removal of secretions. A series of animal and human studies by Unoki et al (2004) demonstrated that chest wall compression moved secretions in a cephalad direction.
Secretion removal techniques
Suction – open/closed.
As critically ill patients are usually intubated, regular pulmonary toilet must be applied. Formerly this was always via the open suction technique: that is disconnection of the endotracheal tube, instillation of a sterile catheter and application of a negative pressure. As the patient did not receive ventilation during this period, an efficient technique in less than 15 seconds was necessary. Most intensive care units now utilize the ‘in-line’ suction technique (closed-suctioning), whereby a sealed catheter is connected to the endotracheal tube and suction is possible without disconnection from the ventilator. This technique has been associated with less risk of desaturation and reduction in lung volume (Cereda et al 2001), fewer arrhythmias, less cardiovascular changes (Lee et al 2001) and less reduction of PEEP (Maggiore et al 2003). However, in pressure-controlled modes of mechanical ventilation, the negative pressure from the suction catheter may trigger ventilator breaths, and the inspiratory flow from the ventilator may force the secretions away from the catheter tip, resulting in fewer secretions being aspirated (Lasocki et al 2006). After suctioning, a lung recruitment technique such as MHI or VHI may be required to minimize the risk of atelectasis induced by the negative pressure suctioning generated by either the open or closed system.
Nasopharyngeal/oropharyngeal suction and mini-tracheotomy.
Nasopharyngeal and oropharyngeal suction have been discussed in detail in Chapter 5. These techniques are often necessary before and during extubation, as well as in attempt to prevent intubation in patients with inefficient coughing efforts or increased secretions.
Increased moisture to airways
Humidification.
Humidification has been discussed in Chapter 5. Humidification is mandatory for patients on mechanical ventilation to reverse some of the adverse effects of intubation such as reduced tracheal mucus velocity and cilial impairment.
A critically ill patient on high concentration of inspired oxygen will also benefit from heated humidification. A heat and moisture exchanger (HME) can be used as an alternative but has been associated with increased circuit dead space and resistance to airflow. It may also be associated with an increased work of breathing in spontaneously breathing patients who are on low levels of respiratory support (Boots et al 2006). The use of HMEs may increase PaCO2 in patients with acute lung injury/acute respiratory distress syndromes (Moran et al 2006). Nebulization with normal saline via the ventilator circuit has been reported to increase the yield of airway secretions (O’ Riordan et al 2006).
Saline instillation.
Direct instillation of normal saline to the endotracheal tube during or prior to suction in an attempt to decrease viscosity of secretions is a frequently used (and yet sometimes controversial) technique. A number of studies have found that this practice results in decreased SaO2 and/or mixed venous saturation (Ackerman & Mick 1998), no increase in secretion yield (Lerga et al 1997) and possible dislodgement/dispersion of microorganisms into the lower respiratory tract (Hagler & Traver 1994). Schreuder & Jones (2004), however, demonstrated increased sputum wet weight and stable arterial oxygen saturation following use of saline when it was combined with chest physiotherapy. It is recommended that instillation of saline should be reserved for patients with excessively tenacious secretions. In addition, the clinician should anticipate a short-term drop in arterial saturation that may require a temporary increase in FiO2.
Positioning
The physiological effects and rationale of positioning have been covered in detail in Chapter 4. Altering the position of a critically ill patient is a powerful tool and may result in both beneficial and adverse effects. Cardiovascular changes associated with positional changes, especially in critically ill patients, should be closely monitored during physiotherapy. An adequate understanding of the pathophysiology of positioning and its predicted effects is essential.
Gravity-assisted positioning.
Traditional gravity-assisted positions (Chapter 5) are often not utilized in intensive care patients as full positioning is often hindered by cardiovascular instability, equipment and lack of patient cooperation. However, evidence suggests that specifically positioning the patient for the affected lobe results in increased expiratory flow rate, better oxygenation, increased sputum clearance and faster resolution without adverse effects on haemodynamic stability (Berney et al 2004, Krause et al 2000).
Prone positioning.
Specific prone positioning for extended periods of time has been advocated as a method to improve oxygenation and lung mechanics in patients with acute lung injury and acute respiratory distress syndrome. There is strong evidence that this method results in improved lung mechanics and oxygenation due to expansion of the collapsed dorsal regions of the lung (Messerole et al 2002). This technique is most useful if used early in the disease process and may also result in increased secretion clearance due to drainage of the collapsed dorsal regions of the lung. Reduction in carbon dioxide with prone positioning is indicative of improved alveolar ventilation and is predictive of better survival (Gattinoni et al 2003).
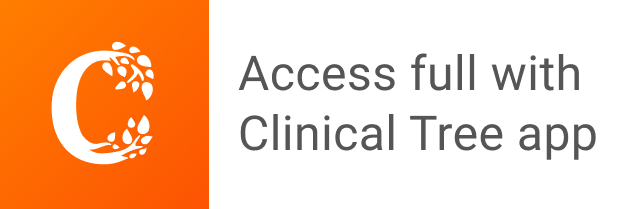