LEARNING OBJECTIVES
Learning Objectives
The student will be able to state normal intrapleural pressures from lung apex to base in the chest of upright and supine subjects, including the effects of gravity.
The student will be able to enumerate the physical factors affecting airway resistance and dynamic lung compliance in vivo.
The student will be able to distinguish normal, restrictive, and obstructive flow patterns and explain peripheral gas trapping by the concept of effort-independent, volume-dependent peak flow rates.
The student will be able to explain how alveolar distension is differentially affected by body position, residual volume (RV), and positive end-expiratory pressure (PEEP) ventilation.
The tissue elastic and surface tension recoil forces that provide inherent compliance to normal lung tissue were reviewed in Chaps. 4 and 5. For excised lungs, these forces can be examined as if they operated independently of constraints placed on lung expansion by bones and muscles of the thorax. However, the chest wall and airways strongly influence lung distension and the work of breathing (Fig. 6.1). Incorporating these factors into a broad model of ventilation will facilitate understanding of the major categories of lung disorders, the obstructive diseases and restrictive diseases.
FIGURE 6.1
The diaphragm is normally the main inspiratory motor during respiration; its caudal excursions create negative pressures that expand the lungs. The intercostals and other accessory muscles enlarge the anterior-posterior and lateral chest diameters. From Fox. Human Physiology, 10th ed.; 2008.
ELASTIC PROPERTIES OF THE INTACT THORAX
The chest wall and diaphragm normally oppose the recoil forces of lung tissue elasticity and surface tension. Thus, the negative PIP at functional residual capacity (FRC) (see Fig. 4.6) represents the equilibrium when these musculoskeletal elements are bowed inwardly by lung recoil. Since this balance is not intuitive, consider a bilateral pneumothorax when PIP = PB (Fig. 6.2). When PIP increases from −5 to 0 cm H2O, healthy lungs recoil toward their minimal volume, while the chest walls spring outward and the diaphragm drops toward the abdominal viscera. Indeed, the volume of the open chest when PIP = PB is about 80% of a subject’s normal total lung capacity (TLC), implying that musculoskeletal elements will oppose all forces attempting to make the chest either larger or smaller than about 80% of TLC.
Thus, an in situ compliance curve for the entire lung-thorax system is the algebraic sum of the compliance curves for each component. Such a composite compliance curve can be obtained by having a subject perform a relaxation pressure-volume maneuver (Fig. 6.3) using a specialized apparatus for whole-body plethysmography (Chap. 16). While simultaneously monitoring PIT in the airway and intraesophageal pressure to approximate PIP, subjects inspire or expire to a series of lung volumes between RV and TLC, and then are locked momentarily at that volume by a valve so they can completely relax their chest wall. Under these conditions, the maneuver begins and the subject’s FRC is empirically defined as that volume when PIT = PB = 0 at the mouthpiece. This FRC also is the volume where inwardly directed lung recoil forces are equal and opposite to outwardly directed forces exerted by the chest wall plus diaphragm.
FIGURE 6.3
Relaxation pressure-volume curves obtained by spirometry combined with whole-body plethysmography. The solid line “lung + chest wall” recorded in the subject’s airways must equal the algebraic sum of compliance curves for excised lungs (right) and the empty thorax (left). Minimal volume = lung volume without alveolar air. From West. Respiratory Physiology; 2005.
When subjects who are at FRC expire toward RV, their PIT is less than PB and a sensation of suction is felt as the thoracic structures are increasingly deviated inward from their preferred resting positions seen with a pneumothorax. Conversely, when the subject inspires to lung volumes above FRC and toward TLC, PIT exceeds PB and a sensation of chest compression develops as lung elastic and tension recoil forces increase. Indeed, the subject’s sensation of chest tightness will maximize at lung volumes >80% of TLC (ie, the size of the thoracic space after a pneumothorax) since the chest wall and diaphragm will also then be exerting inwardly directed positive pressures that augment the positive pressure created by lung recoil.
From this explanation, it becomes apparent that if a subject breathes at lung volumes between FRC and about 80% of TLC, each inhalation to inflate the lungs is achieved in part by utilizing potential energy stored in the chest wall and diaphragm as they were pulled inward during the preceding exhalation. By the same argument, every exhalation uses potential energy stored in the inflated lungs to expel the tidal breath, while simultaneously storing potential energy in musculo-skeletal elements being bowed inward. Thus, it is not accurate to consider normal inhalation to be entirely “active” or normal exhalation to be entirely “passive”. Rather, normal breathing is carefully controlled to oscillate around the midrange of available lung volumes so that inhalation consumes less energy than one might think, but exhalation also costs energy.
CLINICAL CORRELATION 6.1
In contrast to this normal pattern, many patients with obstructive lung diseases (eg, emphysema, asthma) will struggle to exhale fully between breaths. They may become hyper-inflated, still breathing tidally but much closer to their TLC. Their work of breathing is vastly increased, since every inhalation will require distending both the lung tissues and the thoracic elements surrounding them. You can experience this phenomenon by first taking a deep breath, and then resume taking small tidal breaths but without allowing yourself to exhale to FRC each time.
AIRWAY RESISTANCE AND DYNAMIC LUNG COMPLIANCE
To this point, models of static lung and chest wall compliance have been used to estimate resistances to ventilation that are generated by surface and tissue recoil of lung tissue, and by thoracic musculoskeletal elements. However, additional resistance to ventilation also occurs in the airways during flow, only to disappear under no-flow conditions such as FRC. Such dynamic airway resistance is nevertheless a powerful determinant of the pattern, energetic cost, and overall efficiency of ventilation. Thus, dynamic airway compliance measurements are made to directly determine in-line resistances due to the airways. Such measurements have shown that most dynamic airway resistance resides in the upper airways (generations 1-7) because their flow rates tend to be high, their end-to-end pressure changes are large, and their aggregate cross-sectional areas are small. By the same argument, the smaller peripheral airways usually contribute less resistance despite having smaller individual diameters, due to their shorter lengths and their exponentially larger cross-sectional areas (Fig. 6.4).
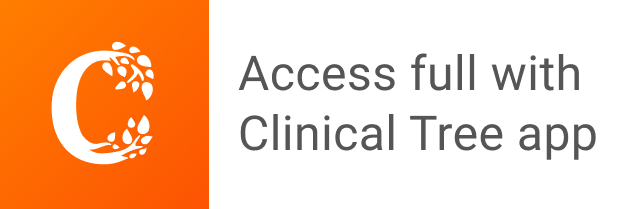