Fig. 2.1
(a) Early steps in heart development. Diagrams of heart development are shown in ventral views. At the earliest stages of heart formation (cardiac crescent), two pools of cardiac precursors exist. The first heart field (FHF) contributes to the left ventricle (LV), and the second heart field (SHF) contributes to the right ventricle (RV) and later to the outflow tract (OT), sinus venosus (SV), and left and right atria (LA and RA, respectively). V ventricle. In (b) a cross section at the level of the dotted line is depicted. Myocardial (cmc) and endocardial (e) progenitors migrate to the midline (m) to form the linear heart tube. They are flanked by endodermal cells (end) and the yolk syncytial layer (YSL) in zebrafish or the endoderm (that invaginates from where the YSL is depicted) in mouse and chick embryos. (An interactive version of the figure can be found at http://pie.med.utoronto.ca/HTBG/index.htm. Images courtesy of F. Yeung, University of Toronto, Canada)
2.1.2 Cardiac Differentiation Is Induced by Signaling Cues from Adjacent Tissues
Mesoderm induction is conserved and regulated by numerous signaling pathways. Some of the key players are: Nodal (a member of the transforming growth factor beta superfamily, TGF-β); bone morphogenetic protein (BMP); the Wnt signaling pathway and fibroblast growth factors (FGF) [1, 9]. Signaling anterior to endodermal cells positively regulate cardiac specification, whereas signaling from the neural plate, somatic and axial mesoderm represses heart formation [10]. Higher levels of Nodal favor cardiac mesoderm induction [11]. BMP function is not clear in the earliest stages of cardiac commitment, although inhibiting BMP signaling seems important to promote the emergence of the cardiac mesoderm [12]. BMP signaling is active in cardiac progenitor cells prior to their differentiation into cardiomyocytes (CMC), and it is continuously required during somitogenesis within the anterior lateral plate mesoderm (LPM) to induce myocardial differentiation in zebrafish. However, at later stages BMP signaling is actively repressed in the ventricle through Smad6a,1 in order to allow for proper chamber differentiation [13]. BMPs seem to be important for expansion of cardiac progenitors, as genetic deletion of the BMP receptor in Mesp1-expressing mesoderm results in major defects in heart formation [14]. Mesp1 expression marks cardiogenic mesoderm (around E6.5 in mouse embryogenesis) and Mesp1−/−; Mesp2−/− double knockout mice lack all mesodermal layer between endoderm and ectoderm [15]. BMP inhibitors noggin and chordin present in the dorsal neural tube and notochord restrict cardiac commitment through Wnt8, Wnt3a inhibition [16]. Wnt antagonists dickkopf1 (Dkk1) and Crescent, inhibit the induction of multiple cardiac foci in adjacent mesodermal cells [17, 18]. FGFs induces cardiac differentiation from the cardiac progenitor cells in early mesoderm [19, 20]. In zebrafish, fgf8−/− mutants fail to initiate proper gene expression of nkx2.5 and gata4 and exhibit severe heart malformations [21].
Specification of splanchnic mesoderm into cardiogenic mesoderm also requires the T-box transcription factor Eomesodermin (Eomes) [22]. In PS stage embryo, Eomes directly activates the basic helix-looped helix transcription factor mesoderm posterior 1 (Mesp1) [23].
T-box factors direct serum response factor (SRF) gene activity early in development and SRF is essential for cardiac regulation of the T-box factors during mesoderm specification [24]. Finally, Tinman (nkx2.5) is the first regulatory gene in any species that was described to be expressed in the precardiac mesoderm and to function in the differentiation of cardiac precursors. In tinman mutants, cardiogenesis is arrested before looping morphogenesis [25]. Nkx2.5 is expressed in the lateral plate mesoderm within the heart field in mouse, frog and avians [26]. In mouse, targeted ablation of Nkx2.5 does not prevent formation of the heart tube, although it does block heart development at the stage of looping morphogenesis, indicating that, if it is critical for the initiation of cardiac cell fate decisions, there must be redundant pathways [27]. The hematopoietic/erythroid cell fate is suppressed via Nkx2.5 during mesodermal fate determination, and that the Gata1 gene is one of the targets that are suppressed by Nkx2.5 [28]. In zebrafish, nkx2.5 and nkx2.7 are redundant during development [29]. Wnt signals from the neural tube normally act to block cardiogenesis in the adjacent anterior paraxial mesendoderm in chick embryos at HH8-9 stage [16].2
2.1.3 Cardiac Crescent Formation
The bilateral precursor pools unite in the midline, cranial to the stomatopharyngeal membrane, forming the cardiac crescent at 3 weeks of human development (E7.5 in mouse) when the embryo is a flat tri-laminar disc [5, 30]. The differentiation of the cardiac crescent into CMCs is dependent on signals derived from the adjacent endoderm. Multiple signals from the craniolateral endoderm are most likely required to induce myocardial specification, and these are balanced by inhibitory factors from other tissues. Candidates that promote specification of myocardial fate include members of the TGF-β, BMP, and FGF [31]. Downstream of these, are members of the MEF2 family expressed in the primary myocardium. In zebrafish, mef2c is expressed in the precardiac mesoderm, followed by mef2A, both apparently limited to the premyocardial and not pre-endocardial cells [32]. GATA transcription factors are also found in the precardiac mesoderm. Gata4−/− mice have an embryonic heart phenotype with more profound the lack of proepicardial cells while, GATA5 induction directs mesoderm-committed progenitors to a cardiac fate and the development of cardiomyocytes [33]. Tbx5 is also essential for cardiac differentiation. It is expressed in the bilateral heart fields but at later stages it is restricted to the future atria [34]. Mutation in human Tbx5 cause the Holt-Oram syndrome, which consists of heart defects and hand bone defects [35]. In zebrafish, tbx5.1 is expressed in cells from the 15-somite stage and marks the bilateral (at both sides of the midline) migrating heart population [36]. Zac1 is strongly expressed in the heart from the cardiac crescent stages and in the looping heart show a chamber-restricted pattern. Zac1−/− mice exhibit ventricular and atrial septal defects, chamber abnormalities and defects in neural tube closure [37].
2.1.4 Linear Heart Tube Formation
At E8 in mouse, or 3 weeks in human gestation, the cardiac crescent fuses at the midline and gives rise to the First Heart Field (FHF) derived linear heart tube. This structure starts beating and subsequently undergoes rightward looping and rapid growth [38]. During the stages that follow, the heart tube expands and additional cells are recruited [5, 39]. Cells from the Second Heart Field (SHF) are added to the heart tube, with preference to the venous and arterial poles [40]. The SHF is located medially to the FHF in the cardiac crescent [5] and the anterior lateral plate mesoderm in zebrafish [41]. SHF cells contribute to the formation of the outflow tract (OFT), the septa, right ventricle, and the inflow of atria [42–45]. SHF is required also in zebrafish for its arterial pole formation [46] and activates FGF signaling pathway [47]. Isl-1 (LIM homeodomain transcription factor) is a marker of SHF, dependent on the Wnt pathway [48]. Isl-1 is required for proliferation and survival of SHF cells as well as for the migration of cardiac progenitors [45]. BMP mediates TGF-β signaling and functions to induce SHF progenitors to differentiate into myocardium [49]. The Notch signaling pathway, negatively regulates β-catenin and keeps progenitors in an undifferentiated state [49]. Loss of β-catenin leads to reduction of Islet-1 cells due to reduced proliferation of these cells. Canonical Wnt signaling3 is involved in the differentiation of the right ventricle, the OFT and the atria [50]. Non canonical- wnt5a, wnt11 ligands are expressed in OFT and are essential for SHF progenitor development and act by restraining the canonical Wnt/β-catenin signaling [48].
2.1.5 Endocardium Development
Cardiac crescent contains both endocardial and myocardial progenitors. The endocardium contributes to the heart tube assembly by directing myocardial migration during heart tube fusion [51]. Studies in zebrafish and chicken embryos showed that endocardial progenitor cells are sequestered within the Heart field [52, 53]. In zebrafish studies, endocardial precursors are restricted in the most ventral zone of the heart field in the early blastula stage [52]. The endocardium of the primary heart tube forms directly from the ventral plexus of endothelial precursor cells, which are in close association with the anterior endoderm. Importantly, endocardial cells have a common origin with myocardial cells, as suggested in quail embryos [54]. QH-1, a quail endothelial marker was first detected in quail embryos at stage 7 [55]. Mouse studies suggested that mesodermal precursor cells in the cardiac crescent maintain fate plasticity and can give rise to both myocardium and endocardium or that the cardiogenic precursors are pre-specified when they migrate from the primitive streak to the cardiac crescent [56]. Endothelial progenitor cells from SHF contribute to the development of endocardium through OFT migration. There is heterogeneity in the origin of endocardium; a part of it derives from vascular endothelial cells [57]. Tal1 is a transcription factor required for endocardial cells migration. In tal1 −/− zebrafish mutants morphogenesis the endocardial cells do not form a single cell layer lining the myocardium, and do not form atrial endocardium. Instead, endocardial cells aggregate at the arterial pole of the heart [58]. GATA5 is required for differentiation of cardiogenic precursors into endothelial endocardial cells [59]. Gata5 is expressed in the endocardium, early in mouse development (E12.5) in atrial endocardium and regulates smooth muscle cell transcription program. NFATC1 interacts with GATA5 to activate endocardial transcription factors [59]. Etv (Etsrp71) transcription factor is expressed in endocardium/endothelium at E8.5 stage and regulates tie2 expression [60]. The transcriptional network involved in endocardial specification is reviewed in [61].
2.1.6 Looping of the Heart
The Heart tube is the first organ that exhibits left- right (L-R) asymmetry. It becomes apparent in chicken embryos at stage HH9 [62] and in zebrafish embryos as cardiac jogging (leftward displacement of the heart cone) at 28 h post fertilization (hpf), and as s-loop at 36 hpf [8]. In humans, s-looping occurs at CS9-10 with the formation of an inner and an outer curvature that gives rise to the ventricles. There is proliferating and differentiating myocardium (secondary/chamber myocardium) from which arise the heart chambers [5]. In zebrafish, the cardiac cone rotates clockwise (venous pole left side, arterial pole midline). In this way, L-R polarity is converted to Dorsal-Ventral axis during heart tube formation, a process dependent on BMP signaling in the LPM, which also directs cardiac progenitor cells migration. For this rotation and migration (cardiac jogging) the Hyaluronan synthase 2 (Has2) expression in cardiac progenitors cells is required [63]. In zebrafish, southpaw, (spaw) a Nodal related gene, is expressed in the left LPM and activates Nodal at the onset of asymmetric morphogenesis in an one eyed pinhead, (oep)-dependent manner at 21-somite stage. Nodal acts in L-R asymmetry by regulating the speed and the direction of CMCs [64]. Nodal signaling directs asymmetric cardiac morphogenesis through establishing and reinforcing laterality information over the course of cardiac development. Cardiac jogging is driven by an asymmetric atrial myocardial migration, influenced by the laterality of gene expression [65]. bmpr1 and bmpr2 are essential for L-R asymmetry in zebrafish, are upstream of pitx2 and spaw and regulate lefty1 [66]. Wnt-GATA4 signaling controls the asymmetric signal propagation from the LPM to the cardiac field. Wnt/β-catenin signaling negatively regulates Gata4 expression in the cardiac field, which subsequently modulates Lefty2 expression in the cardiac primodium. In contrast to the inductive role of Spaw, Wnt/β-catenin signaling imposes a permissive function on the cardiac field, allowing it to respond to asymmetric cues such as Spaw [67]. Sonic Hedgeghog (Shh) in mouse is expressed in the node (the source of mouse left-right asymmetry), the notochord and the gut endoderm [68]. Hedgehog controls L/R asymmetry by the induction of left-side determinants and is required for activation of Gdf1 (Tgf family, upstream of Nodal, lefty1/2, Pitx2) within the node [69].
2.1.7 Chamber Formation
The heart starts functioning and transitions from a linear tube to a looped structure with distinct differentiated chambers. During cardiac looping an inner and an outer curvature of the myocardium are being specified [6, 70]. The outer curvature gives rise to the future ventricles and in the dorso-lateral side of the primary heart tube, two atrial appendices form [6]. The Atrial Natriuretic Factor (ANF) gene is expressed in the working myocardium in the outer curvature of ventricle and atrium of chicken stage HH9 [71]. In zebrafish embryos by 28hpf, sided by the heart tube, in regions supposed to become outer curvatures of ventricle and atrium [72]. The molecular signals in chamber formation are Nppa (anf), the gene encoding ANF and the transcription factors COUP-TFII, Hand1, Irx4 and Irx5, Nkx2-5, Gata4 and members of the T-box gene family. These, in turn, regulate a number of chamber specific genes such as those encoding the gap-junction proteins connexins [73] and as reviewed in [1].
2.1.8 Cardiac Valves and Septa
Cardiac valves originate from endocardial cells at specific positions along the anteroposterior axis of the heart after the heart starts beating. They serve throughout the lifespan of an organism to prevent retrograde blood flow. A number of processes, including Epithelial to Mesenchymal Transition (EMT), lead to the differentiation of valve progenitor cells at the AtrioVentricular (AV) canal from the endocardium [74]. In mice, this process initiates at day (E9.5–10.5) with the formation of cushions by the migration of these mesenchymal cells into the cardiac jelly secreted by the overlying myocardial cells. Highly regulated signaling pathways orchestrate their development. These pathways include Notch, BMP/TGF-β, Wnt/β-catenin, NFATc, VEGF, Erb2/4, has2, microRNAs and others, as reviewed in [75], [76] and chapter 9 of this book. In addition to the above-mentioned signaling pathways, cardiac valve morphogenesis is regulated by the heart function and intracardiac flow dynamics. Endocardial cells at the AV canal as well as the OFT of the heart, experience retrograde blood flow when the heart starts to function and before the valves are formed. Studies in zebrafish embryos, which can survive for several days and develop in the absence of a functional heart beat or blood circulation, revealed the importance of intracardiac flow dynamics in valve morphogenesis [77–80]. Valve cushion mesenchyme differentiates into the fibrous connective tissue of both inlet and outlet valvular leaflets. In addition, the tricuspid valves as well as the septa are partially myocardialized, due to the invasion of cushions by myocardial cells to give a muscular component to the terminally differentiated tissues. Thus, myocardialization appears to be a normal morphogenetic event occurring in most vertebrates, including humans, to give specific cushion structures their mature phenotype. The extracellular cell matrix (ECM) proteins fibrillin and fibulin as well as proteoglycans are thought to be involved in the remodeling of the cushions into valvuloseptal tissue. These molecules accumulate at the cell surface or pericellular matrix of the migrating cushion cells that secrete them and appear to play a role in the final positioning and spacing of cells within the differentiated tissue, reviewed in [74].
2.2 The Signaling Pathways Involved in Cardiac Development Are Responsible for Congenital Heart Diseases
It is no surprise that the above-described complexity of form and function of the heart, leads to an increased incidence of related diseases. A core of conserved transcription factors, including Nkx2.5, GATA4 and Tbx5 are critical in regulating the development of the heart. These transcription factors, as well as their regulatory network (upstream activators and downstream targets) represent a significant percentage of congenital heart diseases (CHD) in humans reviewed in [81]. GATA4 has multiple roles during development in the specification of the myocardium as well as in later stages in AV cushion morphogenesis. In addition, GATA4 is activated during pathologic hypertrophy. Both in the United States and Europe, heart disease (HD) is the leading cause of death and disability and the greatest public health problem. HD is the result of a genetic mutation, congenital diseases, as well as different types of insults in the adult heart, such as coronary artery disease, hypertension and chemotherapy [82]. Although these insults have a different pathophysiological mechanism of action, they all lead progressively to CMCs loss and consequently to the deterioration of heart function. For example, in myocardial infarction, after the initial deprivation of O2 in the myocardium an ischemic cascade is activated with the end result being cell death and its replacement by a collagen rich scar tissue. Scar tissue deposition allows for the initial stabilization of the induced damage, but it is not contractile and thus compromises heart function in the long run. The loss of contractility elicits a hypertrophic response through the activation of signaling pathways like GATA4 but this does not result in an increased number of cardiomyocytes in mammals, that lack the regenerative response capable of replenishing the lost cardiomyocytes. A new research field has emerged, aiming to understand the mechanisms in animal models that maintain the ability to regenerate their hearts and how this could be activated in humans.
2.3 Regenerative Capacity
Regeneration (REG) is the replacement of cells after trauma and results in a partially or fully restored and functional organ. The renewal of the intestinal lining or the maintenance of skin, hair and bone of an organism, are examples of regenerative potential. This natural replacement of cells lost in day-to-day minor and physiological damage, is referred to as homeostatic REG, while the actual REG per se is a unique posttraumatic response. The first paradigm of REG was first observed and reported in Hellenic mythology through the myth of the Titan Prometheus. The myth describes that Prometheus was chained to a rock where each day an eagle devoured his liver, which was growing back. Hundreds of years had passed since the myth of Prometheus until 1768 when Spallanzani reported that amputated salamander limbs were capable to fully regenerate [83]. In the following centuries, more organisms were added to the list of organisms capable to regenerate, among them lizards, frogs and fish. These can regenerate not only their limbs but also different organs.
2.3.1 Heart Regeneration Across Different Species
In mammals, several organs retain their regenerative capacity during adult life. Skin and liver, for example, demonstrate robust examples of mammalian organ REG. Other organs, in the contrary, such as the nervous system and the heart lose their regenerative potential early after birth. However, when hearts of neonatal mice were amputated 1 day after birth, they demonstrated a robust regenerative response completely restoring the damaged ventricle [84]. In addition, neonatal mice retain a regeneration response up to 7 days after birth not only after ventricle amputation but also after coronary ligation, an experimental model closer to the pathophysiology of myocardial infarction [85]. Interestingly, contrary to the long-standing belief that adult human hearts lose their regenerating capacity, there is growing evidence of cardiomyocyte renewal during the lifespan of an adult human. Using the integration of radiocarbon 14C, cardiomyocytes were found to renew at a rate of ~1 % of the entire population per year in a person at the age of 25 years, but this rate drops progressively with the advancement of age [86]. Although the heart of higher adult vertebrates loses its ability to regenerate after an injury, the above studies reveal that regenerative potential is not an evolutionary advantage (Fig. 2.2).
2.3.2 Regeneration in Zebrafish
The urodeles (for example, salamanders) are the champions of heart REG. They can regenerate it even after a 50 % injury [87], by a mitotic response of the preexisting mature cardiomyocytes, which undergo successive cell divisions [88]. Lately, zebrafish has emerged as a novel model with remarkable regenerative capacity and with numerous additional advantages, such as the amenability to in vivo cell biological studies and to forward and reverse genetics approaches [89]. Zebrafish (Danio rerio) is a fish found in fresh water streams from South and East Asia. Zebrafish is a cyprinid and belongs to the greater family of teleosts. Organs such as the kidney, liver, pancreas, spinal cord, CNS, retina and fin can be regenerated in zebrafish after injury [90–92]. The ability of zebrafish to regenerate its heart, was first demonstrated in 2002 when part of the ventricle was amputated and it fully regenerated within 60 days [93]. This unique characteristic of the zebrafish heart it is not a transitory ability but it is preserved throughout its lifetime [94]. The technique of ventricular apex amputation creates initially a thrombus and subsequent activation of the regeneration response [93]. Since then, several techniques have been developed to study heart REG in zebrafish. In cryoinjury-induced damage, a probe that is precooled in liquid nitrogen is applied on the surface of the ventricle, resulting in massive cell death [95, 96]. This type of injury elicits initially an inflammatory response where inflammatory cells invade the injured tissue. In the following days, there is a gradual activation of different type of cells that migrate to the site of injury and initiate the reparative phase with collagen deposition. The cryoinjury method resembles the events that follow a myocardial infarction. In both methods of injury, fully replenished myocardial tissue is achieved after 60 days.
In addition to these surgical methods, genetic approaches have been developed, using transgenic zebrafish that express the bacterial nitroreductase specifically in the heart. Nitroreductase is an enzyme that catalyzes the reduction of the prodrug metronidazole in a cytotoxic product. When zebrafish are exposed to media with metronidazole, cell death is induced in a time and dose dependent manner [97]. Alternatively a heart specific tamoxifen inducible Cre recombinase line was created and crossed with a line capable of expressing diphtheria toxin under the promoter of β-actin upon recombination achieving more than 60 % cardiomyocyte specific death [98]. Finally, ventricular specific embryonic ablation during development showed that atrial cells can transdifferentiate to contribute to ventricular regeneration [99]. In conclusion, genetic methods allow a more global injury of the ventricle in respect to the focally located mechanical injury, thus allowing the study of acute and chronic heart failure mechanisms in regenerative models.
Chemical induction of hypoxia and hypoxia-induced injury is also used to study zebrafish heart REG. Hypoxia refers to the lack of oxygen in the blood and is the hallmark of the ischemia induced heart injury in higher mammals. Since the previously described methods lack the ability to generate an oxygen-deprived environment in the heart tissue, this methodology provides an alternative and a more reliable method to study ischemia induced rather than infarcted injuries [100]. Finally, exposure to aristolochic acid during early development causes a reduction of cardiomyocyte proliferation and heart failure at the larval stage [101]. This model allows the study of progression to heart failure and heart regeneration at early stages, where zebrafish retains a plethora of advantages, including the ability to do large-scale chemical screens.
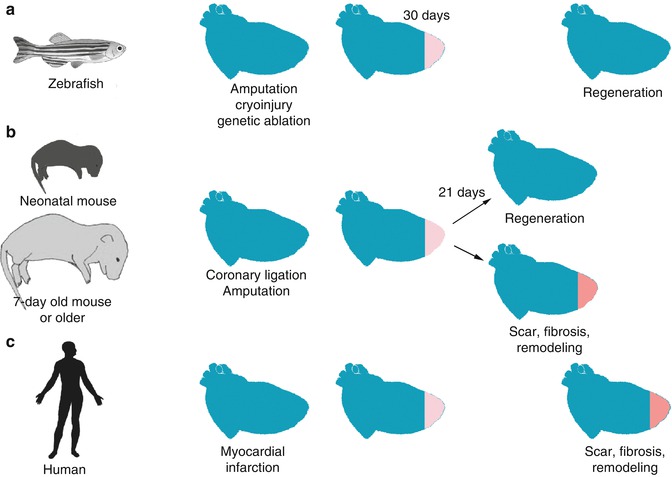
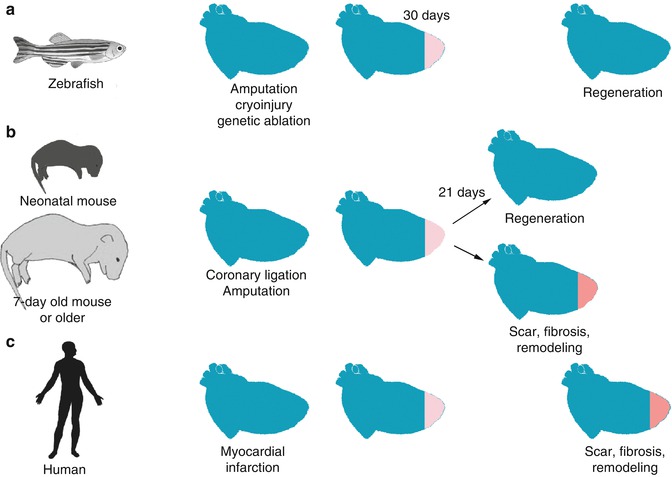
Fig. 2.2
Regenerative capacity is lost in the adult human heart but is maintained in neonatal mouse and zebrafish. (a) Zebrafish maintain their ability to fully regenerate their myocardium within a month after injury. Current experimental models include cryoinjury, ventricular amputation or genetic ablation of myocardial cells. Activated epicardium is necessary for the induction of myocardial proliferation. (b) In neonatal mice, upon ventricular amputation or coronary ligation the myocardium regenerates and is fully functional with no signs of fibrosis. However, this competence is lost after the first week of life. (c) In contrast, in humans upon myocardial injury, a collagenous scar is deposited compromising the myocardial function and initiating a remodeling transcriptional program
2.3.3 Epicardial Cells and Transcriptional Networks Involved in Heart Regeneration
Understanding the mechanisms that are activated during the regenerative response after injury is necessary to be able to re-activate them in humans. It is therefore important to elucidate both the sources of the different types of cells and the relevant signaling pathways. One of the first questions that were addressed was if the sources of the new cardiomyocytes were either undifferentiated progenitor cells or existing cardiomyocytes, which undergo dedifferentiation and proliferation. Amputation experiments in zebrafish showed that undifferentiated progenitor cells initiate REG, in addition to epicardial cells. The activated epicardial cells express FGF receptors and migrate to the site of injury playing a key role to the REG process. Blocking the FGF receptor by expressing negative dominant FGF receptor or treating with an FGFR inhibitor, arrests heart REG. Coronary neovascularization is impaired because the endocardial cells fail to undergo endothelial to mesenchymal transition [102]. Epicardial cells also regulate the extracellular matrix at the injury site, by enriching it with fibronectin. Fibronectin is a key component of the extracellular matrix and it is previously associated with fibrosis after cardiac damage in adult mammals [103, 104]. A transgenic zebrafish that expresses under a heat-shock inducible promoter a truncated, not functional fibronectin after amputation of the ventricle was used to show that defects in fibronectin deposition are associated with impaired REG and accompanied by increased fibrosis [105]. Genetic fate mapping studies demonstrated that pre-existing cardiomyocytes are capable of dedifferentiation and proliferation after resection of zebrafish heart [106, 107]. Furthermore, not only de-differentiation and proliferation of existing CMCs is essential for heart REG but also their migration to the injury site. Pharmacological blocking of a chemokine receptor expressed in CMCs especially after injury arrests regeneration after amputation of the ventricle [108]. The Notch signaling pathway is highly conserved across species and plays a key role in cell-to-cell communication regulating cell fate decisions during embryogenesis [109]. In particular, Notch signaling is involved in the development both of the myocardium-ventricular morphogenesis-and the formation of the valves [110]. Interestingly, the Notch signaling pathway has also been implicated in the REG process after ventricular amputation of zebrafish heart [111]. In particular, different components of the Notch signaling pathway are upregulated before REG takes place, designating an activation of embryonic genetic program during injury.
< div class='tao-gold-member'>
Only gold members can continue reading. Log In or Register a > to continue
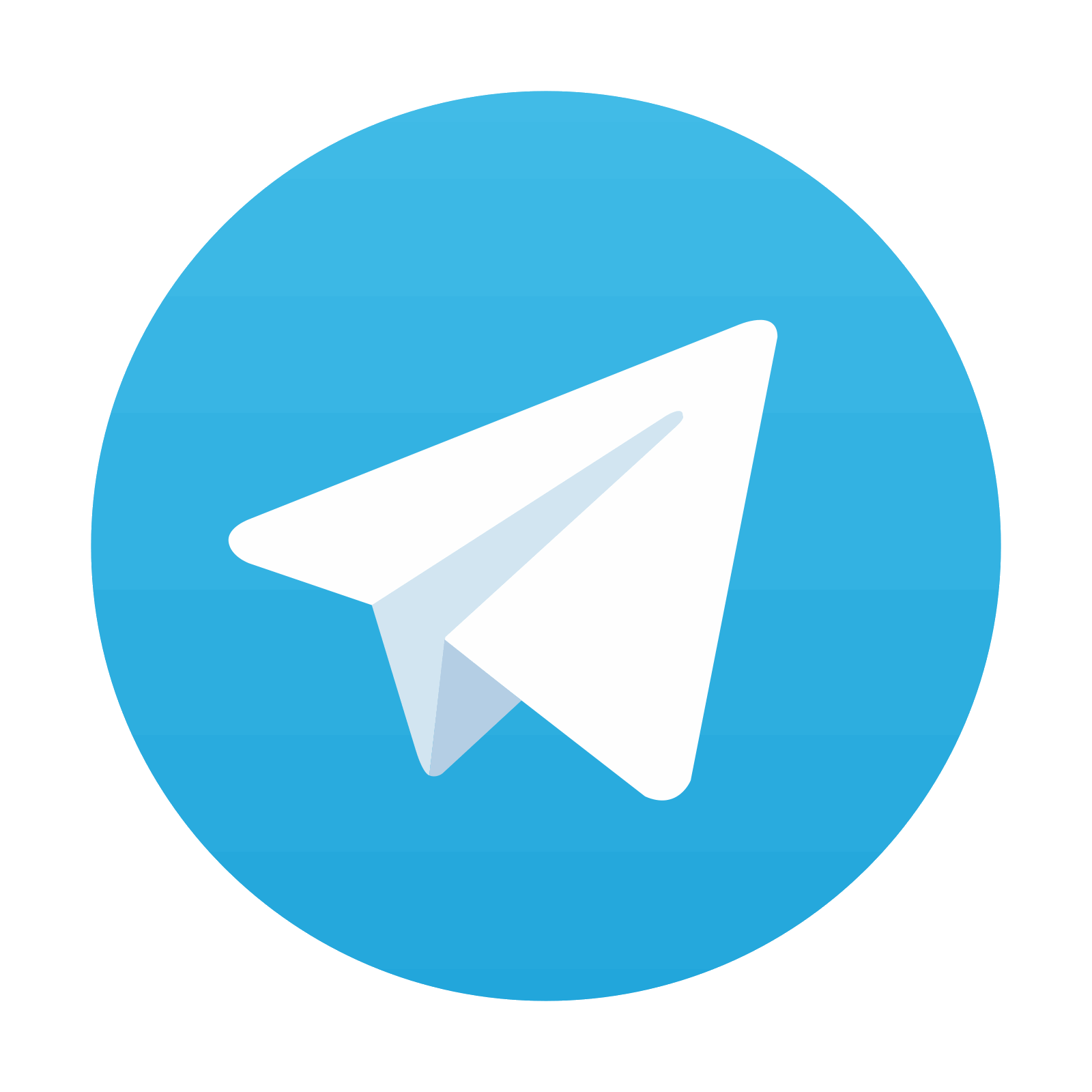
Stay updated, free articles. Join our Telegram channel
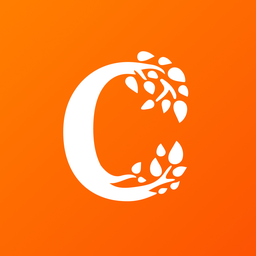
Full access? Get Clinical Tree
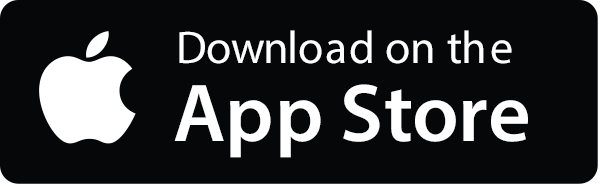
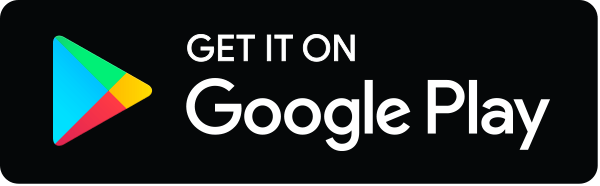