Abstract
Infants and children with congenital and acquired heart disease frequently present with nonspecific complaints, and therefore it is essential to have a high index of suspicion for a cardiac cause. This is particularly true for a neonate presenting with severe cyanosis or cardiovascular collapse. A thorough knowledge of pediatric cardiac physiology and O 2 delivery are required to accurately evaluate and effectively stabilize these patients. The safe transport of patients with known or suspected cardiac disease requires a clear understanding of the transport environment’s unique features and an appreciation of the challenges that these may pose. Effective triage and communication is essential to assessing the patient’s severity of illness and clinical stability before transport and to determining what interventions and/or therapies will be needed on arrival to the referring facility, during transport itself, and immediately upon return to the receiving institution.
Key Words
Oxygen delivery, Pulmonary blood flow, Systemic blood flow, Prostaglandin E 1 , Transport, Triage, Elective intubation
Infants and children with congenital and acquired heart disease can present with a myriad of nonspecific findings, including poor feeding, cyanosis, tachypnea, respiratory distress, shock, and altered mental status. A thorough knowledge of cardiac physiology is required to accurately evaluate and effectively stabilize these patients, including an understanding of the typical age and pattern of presentation.
The diagnosis of ductal dependent congenital heart disease (CHD) has continued to evolve over the last decade. Although nearly universal screening ultrasound examinations have increased prenatal diagnosis of critical CHD (those requiring intervention within the first year of life), the majority of these patients continue to be diagnosed postnatally. For this reason, most US states now mandate that pulse oximetry screening for critical CHD be completed before a newborn is discharged from the hospital. Such screening consists of a single measurement in the lower extremity after 24 hours of age. In the United States it is estimated that pulse oximetry screening will detect almost 1200 neonates with critical CHD and prevent 20 neonatal deaths each year.
On the other side of the spectrum, more patients are surviving into adulthood with CHD. Although many of these patients have minimal or no residual defects, patients with more complex lesions may have progressive evolution of their altered physiology or residual disease, thus requiring an understanding of the long-term complications of residual defects and surgical repairs.
Goals of Management
The primary goal in caring for all infants and children with cardiac disease is to ensure adequate oxygen delivery (DO 2 ). The heart must provide sufficient blood flow (cardiac output) to preserve organ function. The amount of blood flow required is dependent on tissue oxygen demand and blood oxygen supply. Conditions that increase demand, such as elevated metabolism from fever or infection, or that decrease supply, such as hypoxemia or anemia, can affect the amount of cardiac output required to sustain normal organ function. Patients with heart defects may have difficulty providing adequate cardiac output if they have inefficient pump function (e.g., intracardiac or extracardiac shunts, valvular insufficiency, arrhythmias) or depressed pump function (e.g., diminished ventricular contractility). Further, conditions of the vasculature, such as hypovolemia or decreased peripheral vascular resistance, may affect the ability of the heart to generate adequate cardiac output. To effectively manage patients with inadequate DO 2 , it is critical to understand the basic factors that constitute it.
DO 2 is a function of systemic cardiac output and arterial O 2 content. Because DO 2 normally far exceeds the amount of O 2 actually consumed during periods of homeostasis, evidence of inadequate DO 2 can be assessed by the amount of O 2 that is extracted during circulation. Fractional O 2 extraction represents the difference between the O 2 content of the arterial versus venous blood. Practically, it can be measured as arterial oxygen saturation (SaO 2 )−central venous oxygen saturation (SvO 2 )/SaO 2 , with less than 0.3 representing normal extraction and greater than 0.5 demonstrating inadequate delivery. As DO 2 falls below the critical threshold, aerobic metabolism can no longer be maintained. Anaerobic metabolism will generate lactic acid as a by-product. Therefore measurement of acidosis or lactate can provide a very useful measure of the severity and duration of inadequate DO 2 .
Understanding the components of DO 2 provides the opportunity to augment supply. Cardiac output is directly related to heart rate and stroke volume. Stroke volume is dependent on preload, afterload, and myocardial contractility. Both pulmonary blood flow (Q p ) and systemic blood flow (Q s ) are determined by these fundamental forces. In the patient with two ventricles, ventricular interdependence, or the effect of one ventricle on the other, may play a role in pulmonary or systemic blood flow. In some situations the pericardium and restriction by the pericardial space may also play a role in cardiac output by altering preload.
Oxygen content is primarily a function of hemoglobin concentration and arterial oxygen saturation. Thus patients who are chronically hypoxemic can improve DO 2 at any given cardiac output by maintaining a higher hemoglobin concentration. Arterial oxygen saturation may be affected by pulmonary parenchymal abnormalities with increased ventilation/perfusion (V/Q) mismatch or by the mixing of systemic and pulmonary venous return. Low mixed venous oxygen content contributes to desaturation and suggests increased oxygen extraction due to inadequate DO 2 . This in turn is due either to systemic cardiac output that is insufficient to meet metabolic needs or to inadequate hemoglobin concentration.
Presentation
Many infants and children with congenital heart defects and heart disease will present to health care providers in a nontertiary care center or one that does not have specialized pediatric cardiac care or intensive care units. These patients may have a known congenital cardiac lesion or may have an unknown or an evolving cardiac process. Recognition and diagnosis of cardiac disease in these patients can be challenging due to their complex physiology, age-dependent presentation, and nonspecific clinical findings and may frequently be misdiagnosed as sepsis or respiratory failure. It is essential that an infant or child who presents with shock, acidosis, or respiratory insufficiency due to a cardiac cause is quickly recognized and stabilized. A thorough understanding of the anatomy and pathophysiology of the congenital cardiac lesions and their impact on DO 2 is necessary to help direct initial stabilization, transport, and subsequent management of such patients.
CHD generally can be categorized as cyanotic or acyanotic. Cyanotic heart disease is caused by insufficient blood flow to the lungs or ineffective mixing of oxygenated and deoxygenated blood in parallel circulations. Acyanotic heart disease is due to (1) inadequate systemic blood flow due to obstruction either into or out of the left ventricle (LV), (2) myocardial dysfunction, or (3) maldistribution of pulmonary blood flow.
Cyanotic Heart Disease
Cyanosis occurs in patients with inadequate Q p or those in whom the pulmonary and systemic circulations occur in parallel with inadequate mixing of oxygenated and deoxygenated blood. Neonates whose Q p is dependent on a patent ductus arteriosus (PDA) may present hours to days after birth, with severe hypoxemia and acidosis as the ductus closes. Decreased Q p may be due to obstructed flow from the inlet of the pulmonary ventricle (e.g., tricuspid atresia) or from its outlet (e.g., severe pulmonary stenosis, pulmonary atresia [PA] with intact ventricular septum, or tetralogy of Fallot). The PDA allows blood to bypass the level of obstruction. An atrial or ventricular septal defect is also required to “decompress” the systemic venous return to the systemic side of the circulation and allow mixing of oxygenated and deoxygenated blood. The blood in the systemic ventricle therefore consists of desaturated systemic venous blood (via the septal defect) and a smaller volume of saturated pulmonary blood depending on the degree of pulmonary obstruction (Q p :Q s <1). Although Q s may initially be normal, with ductal closure systemic DO 2 falls rapidly due to both decreasing Q p :Q s and greater O 2 extraction. This results in anaerobic metabolism, acidosis, and myocardial dysfunction.
Neonates may also present with significant cyanosis in the presence of inadequate mixing of parallel pulmonary and systemic blood flow as is seen with d-transposition of the great arteries (d-TGA) or with obstructed pulmonary vein outflow as seen with total anomalous pulmonary venous return (TAPVR). Patients with d-TGA with intact ventricular septum will present at birth with severe cyanosis but with preserved Q s . Acidosis and myocardial dysfunction will develop over time as O 2 saturations fall, though the rapidity will depend on the presence and size of an atrial shunt to provide mixing. Patients with obstructive TAPVR also present with cyanosis but may demonstrate significant respiratory insufficiency due to pulmonary hypertension with increased transmural pressure across the pulmonary vasculature and pulmonary edema formation.
The differential diagnosis of a neonate who presents with severe cyanosis must include the possibility of a ductal dependent pulmonary circulation with a closing ductus arteriosus. For neonates with suspected CHD the initial evaluation should include the following:
- •
Four-extremity blood pressure measurements (e.g., upper and lower limb systolic blood pressure difference >10 mm Hg usually indicates aortic arch abnormalities).
- •
Preductal and postductal oxygen saturation measurements (oxygen saturation as measured by pulse oximetry [SpO 2 ]) to identify differential cyanosis (i.e., preductal SpO 2 > postductal SpO 2 ) and reverse differential cyanosis (i.e., postductal SpO 2 > preductal SpO 2 ). A preductal-postductal SpO 2 difference of more than 10% usually indicates ductal dependent CHD. Reverse differential cyanosis is usually observed in TGA but was also described in supracardiac total anomalous pulmonary venous connection.
- •
Hyperoxia test to differentiate between CHD and non-CHD (e.g., SpO 2 <85% on both room air and 100% oxygen usually indicates CHD).
Other causes of central cyanosis in a neonate should be evaluated concurrently with initiating prostaglandin E 1 (PGE 1 ) while establishing a definitive diagnosis. These causes include pulmonary causes such as persistent pulmonary hypertension, congenital diaphragmatic hernia, pneumothorax, or pleural effusion, as well as central nervous system depression due to perinatal asphyxia, neurologic disorder, or maternal sedation. In addition to four-extremity blood pressure measurements, preductal and postductal saturation levels, and hyperoxia testing, the diagnosis can be further elucidated by physical examination; chest x-ray examination to evaluate cardiac size, position, and shape and vascular markings; electrocardiogram to assess ventricular hypertrophy, conduction defects, or arrhythmia; and arterial blood gas measurement to assess gas exchange and acidosis. Ultimately, an echocardiogram will be required but will not always be available on presentation.
Acyanotic Heart Disease
Children with acyanotic heart disease will present emergently when Q s is inadequate. This may represent (1) ductal dependent systemic circulation with obstructed flow into or out of the LV (e.g., mitral stenosis, aortic valve disease, aortic coarctation), (2) myocardial dysfunction (e.g., cardiomyopathy, anomalous coronaries), or (3) maldistribution of pulmonary blood flow with left-to-right shunts (e.g., ventricular septal defect [VSD]). These lesions result in inadequate systemic DO 2 either by actual obstruction to flow through the left heart or by excessive Q p with a functional decrease in blood flow to the systemic circulation. As the pathophysiology for each of these defects worsens, the ability of the lungs to oxygenate blood is impaired due to increased intravascular and extravascular lung water and elevated pulmonary vascular resistance (PVR).
Ductal Dependent Systemic Circulation.
Limitation to Q s can occur at a number of levels along the left ventricular outflow tract (i.e., hypoplastic left heart syndrome, interrupted aortic arch, critical coarctation of the aorta). Systemic blood flow in these patients, as in those with ductal dependent pulmonary blood flow, depends on flow through a PDA to bypass the level of obstruction, as well as a septal defect to allow adequate egress from the pulmonary circulation. The neonate, if not diagnosed prenatally or before discharge home, is likely to present following ductal closure with progressive increase in LV afterload, a decrease in myocardial function, and acidosis due to inadequate DO 2 . Clinically this is manifested by poor perfusion with decreased pulse rates in the lower extremities and pulmonary edema due to increased afterload to the pulmonary vascular bed and transudation of fluid. Ultimately, complete closure of the ductus will cause profound cardiovascular collapse.
As in the case of severe cyanosis, the differential diagnosis of a neonate who presents with circulatory collapse must include the presence of a ductal dependent lesion with a closing ductus arteriosus. This evaluation should also include four-extremity blood pressure measurements, preductal and postductal saturation levels, and a hyperoxia test in addition to physical examination, chest x-ray examination, electrocardiogram, and cultures. This presentation is frequently mistaken for severe sepsis. Although this diagnosis must also be considered and empirically treated, the use of PGE 1 should not be delayed while confirming the diagnosis.
Myocardial Dysfunction.
Inadequate O 2 delivery due to significant myocardial dysfunction may be due to cardiomyopathy, coronary anomalies, arrhythmias, or progressive cardiac dysfunction due to other lesions. Cardiomyopathy may represent primary defects due to mitochondrial or metabolic disorders or to inflammatory heart diseases such as myocarditis. Patients with structural congenital heart defects may also develop myopathic changes in the heart due to volume or pressure overload. Significant myocardial dysfunction may present as severe hypoxemia, acidosis, and/or ventricular failure. Specific clinical signs depend on age and cause. Neonates and infants are more likely to present with failure to thrive and feeding intolerance, diaphoresis, tachycardia, mottling, and respiratory distress. In addition to these findings, infants with cardiomyopathy due to anomalous origin of the left coronary artery from the pulmonary artery (ALCAPA) may present with extreme irritability, particularly during feeds due to intermittent cardiac ischemia caused by coronary steal with the drop in PVR over the first weeks of life. The coronary anatomy of any infant who presents with cardiogenic shock of unknown cause must be assessed to rule out the presence of ALCAPA or other anomalies of the left coronary artery. Older children tend to present with more overt signs of LV failure, including tachycardia, decreased peripheral perfusion, pulmonary edema, dyspnea, and abdominal pain. These ultimately may progress to cardiogenic shock.
Maldistribution of Pulmonary Blood Flow.
Infants with large left-to-right shunts are likely to develop maldistribution of pulmonary blood flow or pulmonary overcirculation (Q p :Q s >1). As PVR decreases after birth, the pressure gradient across the shunt increases and augments the degree of shunting. These patients are likely to present at greater than 1 month of age with tachypnea, increased work of breathing, hepatomegaly, diaphoresis, and poor feeding. The increased metabolic requirement associated with excessive respiratory and cardiac work also leads to poor weight gain and typically a history of failure to thrive. If Q p is markedly increased, particularly with left-to-right shunts distal to the tricuspid valve, such as a large VSD or aortopulmonary window, Q s will decrease. This occurs both by overcirculation of the pulmonary vasculature with reduced ejection of blood from the systemic ventricle and by an overall decrease in myocardial function due to LV volume overload. Each of these mechanisms results in pulmonary congestion with increased V/Q mismatch. Over time, increased Q p leads to a series of pulmonary microvascular changes that first produce reversible pulmonary vasoconstriction and later fixed pulmonary vascular disease.
Initial Stabilization and Management
The initial stabilization and management of infants and children with cardiac disease must focus on establishing adequate DO 2 . To do this effectively there must be adequate and balanced output to both pulmonary and systemic circulations. Manipulating cardiac output and O 2 carrying capacity may also be necessary depending on the nature and severity of the lesion and the degree to which the lesion has affected myocardial function. In addition to cardiac considerations, initial stabilization should take into account abnormalities of other organ systems that occur as comorbid conditions or may be due to the effects of inadequate DO 2 on end-organ function.
Ensure Adequate Pulmonary and Systemic Output
Intravenously administered PGE 1 dilates the ductus arteriosus to provide Q p or Q s to neonates with ductal dependent lesions. An infusion of PGE 1 should be initiated for any clinically unstable neonate with severe cyanosis or cardiovascular failure to reestablish flow. This should be done before confirming the presence of a ductal dependent lesion. Patients who may benefit from early PGE 1 initiation include (1) those with right-sided cardiac lesions who need to maintain Q p (tricuspid atresia, PA, tetralogy of Fallot with PA, Ebstein anomaly), (2) those with left-sided cardiac lesions that need to maintain Q s (hypoplastic left heart syndrome, critical aortic coarctation, interrupted aortic arch), and (3) those with parallel circulations (d-TGA) to augment effective mixing of circulations, though this is likely to be insufficient without an atrial septal defect (ASD) of adequate size. However, PGE 1 administration may be not helpful in d-TGA with intact ventricular septum with a small ASD because there is limited mixing and critical aortic stenosis because the ductus is distal to the area of obstruction and the LV is still subjected to outflow; PGE 1 administration may be relatively contraindicated for patients with obstruction of the blood flow into or out of the left atrium (e.g., TAPVR with obstruction). In those conditions the benefits of PGE 1 outweigh the risks, and PGE 1 should be started pending definitive diagnosis. Consideration must also include the serious side effects of PGE 1 infusion, which may be dose related. Up to 38% of patients may develop one or more adverse reactions, the most common being apnea (18%), hypotension (13%), and fever (11%). The starting dose for the PGE 1 infusion is 0.01 to 0.03 mcg/kg/min, though this should be initiated at a higher dose (0.05 to 0.1 mcg/kg/min) if the ductus has closed and the patient is in extremis. To decrease the risk of side effects, once patency is established, the dose should be minimized if possible. In addition to the presence of a PDA, lesions with obstructed Q p or Q s also require a site of mixing of Q p and Q s , typically an ASD. As described earlier in the case of a minimal ASD, reestablishing patency of the ductus is unlikely to provide adequate mixing of the circulations, and an atrial septostomy may be required. This should be considered before transport so that the accepting team can have the necessary personnel and equipment present on the infant’s arrival.
Once ductal patency has been restored, cardiac output must be optimized and balanced between pulmonary and systemic circulations. In the absence of a fixed obstruction the distribution of total cardiac output to the pulmonary and systemic circulation is determined by the relative resistance of each vascular bed ( Table 42.1 ). PVR is affected by pH, pCO 2 , pO 2 , lung volume, noxious stimuli, hematocrit, and a number of medications. Patients with excessive Q p and consequent low DO 2 to the systemic circulation can be managed by increasing PVR to decrease Q p and increase Q s . Conversely, decreasing PVR will increase Q p and decrease the relative flow to the systemic circulation. Therefore significant care should be taken with overventilation or oxygenation of patients with ductal dependent systemic circulation. In discussing this with the transport team, it is essential that the communication be concise and goal directed.
↑PVR | ↑ Systemic Ventricular Afterload |
---|---|
|
|
↓ PVR | ↓ Systemic Ventricular Afterload |
|
|
Similarly, alterations in PVR can help improve hemodynamics in other cardiac lesions. Minimizing PVR can decrease RV afterload to encourage more forward flow out of the RV to increase LV preload and possibly cardiac output as long as the LV is compliant. Manipulation of PVR also provides some control over intracardiac shunting and effective cardiac output. Shunting through large intracardiac shunts is affected by the relative resistances of the downstream circuits. In the case of an ASD the compliance of the ventricle will determine its filling pressure and therefore the pressure gradient across the ASD. Shunt flow across an ASD is typically left to right because the right ventricle (RV) is typically more compliant than the LV. However, in newborn infants with an ASD the RV may be relatively stiff or noncompliant, and the direction of shunt flow may be right to left, resulting in some arterial oxygen desaturation. For VSDs and extracardiac shunts the downstream resistance is provided by the systemic and pulmonary vasculature. Decreased PVR will increase Q p :Q s and reduce effective systemic perfusion. In most cases the PVR is substantially less than the systemic vascular resistance (SVR), and shunt flow will be toward the pulmonary circuit.
Stabilize Hemodynamics
Myocardial function is determined by both the contractile function and the loading conditions of the heart. The general approach to improving cardiac output is to augment contractility with inotropic support, to optimize preload with careful titration of fluid resuscitation or diuretic therapy, and/or to minimize afterload of the RV and/or LV with vasodilators or by altering PVR as described earlier. Additional support of LV function can be achieved with positive pressure ventilation by decreasing wall stress with resultant decrease in the afterload on the LV. The adequacy of DO 2 can also be improved by minimizing O 2 consumption with temperature control, sedation, and mechanical ventilation.
Myocardial contractility is controlled by the presence of intracellular calcium and therefore can be improved by increasing ionized calcium or infusing inotropic medications, such as catecholamines or phosphodiesterase inhibitors. Calcium supplementation plays an essential role in augmenting LV function in children. The underdeveloped sarcoplasmic reticular system in the neonatal myocyte causes the myocardium to be more dependent on extracellular calcium concentration than the adult myocardium. Because intracellular calcium plays a central role in myocardial contractility in neonates, blood levels of ionized calcium should be normalized to augment stroke volume. Infants with chromosome 22q11 deletions (velocardiofacial syndrome, DiGeorge syndrome) are particularly susceptible to low calcium levels.
Catecholamines, or beta-adrenergic agents, represent a class of agents that provide varying effects on contractility, heart rate, and vascular resistance depending on the specific receptors stimulated. The more frequently used agents include epinephrine, dopamine, dobutamine, and isoproterenol. Epinephrine is typically used at a dose of 0.03 to 0.3 mcg/kg/min, with predominant beta-1 effects and increased contractility at lower doses. At higher doses, alpha-1 effects predominate with vasoconstriction. Dopamine is used at doses of 3 to 10 mcg/kg/min, though its use may be limited because it is associated with a greater incidence of arrhythmias, as well as increased PVR at higher dosing. Dobutamine is an effective positive inotropic agent but frequently produces unacceptable degrees of tachycardia. Isoproterenol is used predominantly for its chronotropic effect. Inotropic drugs increase contractility by increasing cytosolic Ca 2+ concentration, which may also impair relaxation of the heart, decrease ventricular compliance, and limit preload. In addition, augmenting inotropy will increase myocardial energy utilization. Milrinone is an inotropic agent that works through an alternative mode of action. As a phosphodiesterase-3 inhibitor, it potentiates the action of cyclic adenosine monophosphate (cAMP) to provide inotropic effects, as well as vasodilation. Data suggest that milrinone minimizes low cardiac output syndrome in some postoperative patients. Milrinone may serve an important role in patients with decompensated heart failure and preserved blood pressure by reducing afterload, particularly given that beta-adrenergic receptors are desensitized in these patients, resulting in a blunted response to catecholamines. It should be remembered, however, that the half-life of milrinone is relatively long at 2 to 3 hours, and its use can cause persistent hypotension and should be used in caution with patients with renal insufficiency. Vasopressor agents such as norepinephrine and vasopressin that increase afterload should be used with great caution in patients with poor myocardial function. These agents do, however, serve an important role in increasing diastolic blood pressure to maintain myocardial perfusion, as well as in the treatment of severe vasoplegia with hypotension due to sepsis or systemic inflammation.
Preload, or the amount of blood that distends the ventricle before contraction, determines the effectiveness of contraction as described by the Frank-Starling law. The impact of preload on output will depend on the compliance of the ventricle. In a compliant ventricle, enhancing preload will increase stroke volume and cardiac output. However, in a poorly compliant ventricle, augmenting preload will negatively impact the strength of contraction, elevate venous pressure, and generate pulmonary edema. The neonatal myocardium is stiffer than that of older children; therefore it both requires a greater than normal preload to maintain output and is less tolerant of excess preload. An infant presenting with congestive heart failure, pulmonary edema, and a stable systemic blood pressure may respond to diuretics to reduce ventricular wall stress and relieve pulmonary edema without compromising ventricular output. However, an infant with a myopathic ventricle presenting with hypoperfusion, hypotension, and acidosis is more likely to require carefully titrated fluid administration to optimize preload and augment cardiac output. Chest x-ray examination and liver size can aid in determining fluid and diuresis requirements. When titrating fluid, careful monitoring of central venous pressure (CVP) is crucial because small changes in volume can cause overdistention in a noncompliant ventricle. In the absence of a CVP, which is typical on transport, we recommend administering smaller-volume boluses (5 mL/kg to 10 mL/kg) with frequent reassessment of the effectiveness of the fluid bolus(s). If there appears to be a positive response with a reduction in heart rate and/or improved blood pressure and perfusion, volume augmentation of preload should continue. However, if there is a worsening of vital signs and/or signs of fluid overload, one should consider stopping volume augmentation and administer diuretics instead.
Reducing afterload may improve myocardial function by decreasing ventricular wall tension and myocardial oxygen consumption and improving stroke volume. Afterload can be optimized by using agents that vasodilate (nicardipine, nitroprusside, milrinone, dobutamine), as well as by avoiding medications (high-dose dopamine, epinephrine, norepinephrine) or situations (pain, agitation) that raise SVR. Patients with left-to-right shunts and LV volume overload have shown improved function with cautious reduction of systemic afterload. Children with LV outflow tract obstruction and pressure overload, such as severe aortic stenosis, may have massively increased, fixed afterload. Administering vasodilators in these patients will not increase Q s but rather may cause shock, myocardial ischemia, or life-threatening arrhythmias. In this situation, afterload reduction is accomplished by relief of the fixed obstruction using surgical or cardiac catheterization techniques.
Mechanical ventilation may be needed to support respiratory failure and decrease O 2 utilization in the case of poor DO 2 . In addition, positive pressure ventilation impacts preload, contractility, and afterload of the right and left ventricles to directly alter cardiac output. During spontaneous ventilation, inspiration generates negative intrathoracic pressure, which enhances venous return to the heart. The increase in preload to the RV augments stroke volume. Positive pressure, on the other hand, increases intrathoracic pressure and reduces preload to the RV. This will be further accentuated by other factors that impact preload, such as hypovolemia or sepsis. Mechanical ventilation augments LV output primarily by altering its transmural pressure. The change in pleural pressure from negative to positive decreases the transmural pressure and lowers the afterload against which the LV must pump. Although this may have minimal impact on a normally functioning myocardium, it can provide significant support to a dysfunctional LV.
Changes in lung volumes also can impact RV afterload by altering PVR. Pulmonary vasculature resistance is determined by the resistance of the alveolar and extraalveolar vessels. When the lung is hyperinflated beyond functional residual capacity (FRC), the small alveolar vessels become compressed, and PVR is increased. Conversely, hypoventilation (with resultant atelectasis) distorts and collapses the larger extraalveolar vessels and increases PVR. Further, atelectasis may also decrease alveolar pO 2 and cause hypoxic pulmonary vasoconstriction. Therefore maintaining lung volume at FRC without overdistention or atelectasis results in optimal PVR. Because minor changes in lung volume and ventilation can have significant effects on hemodynamic function, transitioning from negative pressure to positive pressure ventilation during resuscitation can significantly destabilize a patient, particularly in the presence of hypovolemia or vasodilation from sedative agents or sepsis. Preload should be optimized in such patients to compensate for increased intrathoracic pressure with initiation of positive pressure ventilation. These effects can be exacerbated in patients with significantly decreased cardiac function. In such cases it is prudent to be prepared for cardiovascular collapse, including the ability to rapidly mobilize extracorporeal membrane oxygenation (ECMO) support if readily available.
Transport
The approach to transporting neonates and children with suspected or known heart disease requires not only thorough knowledge of pediatric cardiac pathophysiology and strong clinical skills, but also clear understanding of the transport environment’s unique features and thoughtful appreciation of the significant heterogeneity that characterizes the field of critical care transport.
Transport Hazards
By virtue of its mobile and underresourced nature, transport is a hostile environment with a multitude of potential hazards that can affect both the patient and the crew: noise, vibration, and poor lighting; exposure to gravitational forces; extreme heat/cold with alterations in thermoregulation; changes in barometric pressure (leading to hypoxia and gas expansion with altitude); limited resources (e.g., personnel, equipment, supplies, therapies); difficulties monitoring patient status; and communication and interpersonal challenges. The safe completion of every transport is contingent upon thorough assessment of these possible risks.
Overview of Critical Care Transport
Critical care transport (CCT) is a unique discipline whose goal is to take critical care services to the patient and rapidly begin advanced care. CCT borrows the use of ambulances, stretchers, and monitors from the prehospital environment and adds critical care–trained personnel and specialized equipment (e.g., isolette, ventilator, intraaortic balloon pump, ECMO pump).
Each CCT has its own variables related to the patient (e.g., age, weight, comorbidities); disease process (e.g., severity of illness, complications, treatments); referring and receiving institutions (e.g., resources, interfacility distance); time of day, geographic and environment conditions (e.g., inaccessible area, traffic, weather); and the transport team’s resources (e.g., personnel, vehicles, equipment, medications, supplies) and communication skills (e.g., interactions with referring staff, patient, family).
Transport Modes
Mode of transport (ground versus air) is determined based on resource availability, transport specifics, and the characteristics of each transport vehicle: ground, rotor-wing, and fixed-wing ambulance ( Table 42.2 ).
Transport Vehicle | Advantages | Disadvantages | Comments |
---|---|---|---|
Ground ambulance |
|
|
|
Rotor-wing ambulance |
|
|
|
Fixed-wing ambulance |
|
|
|
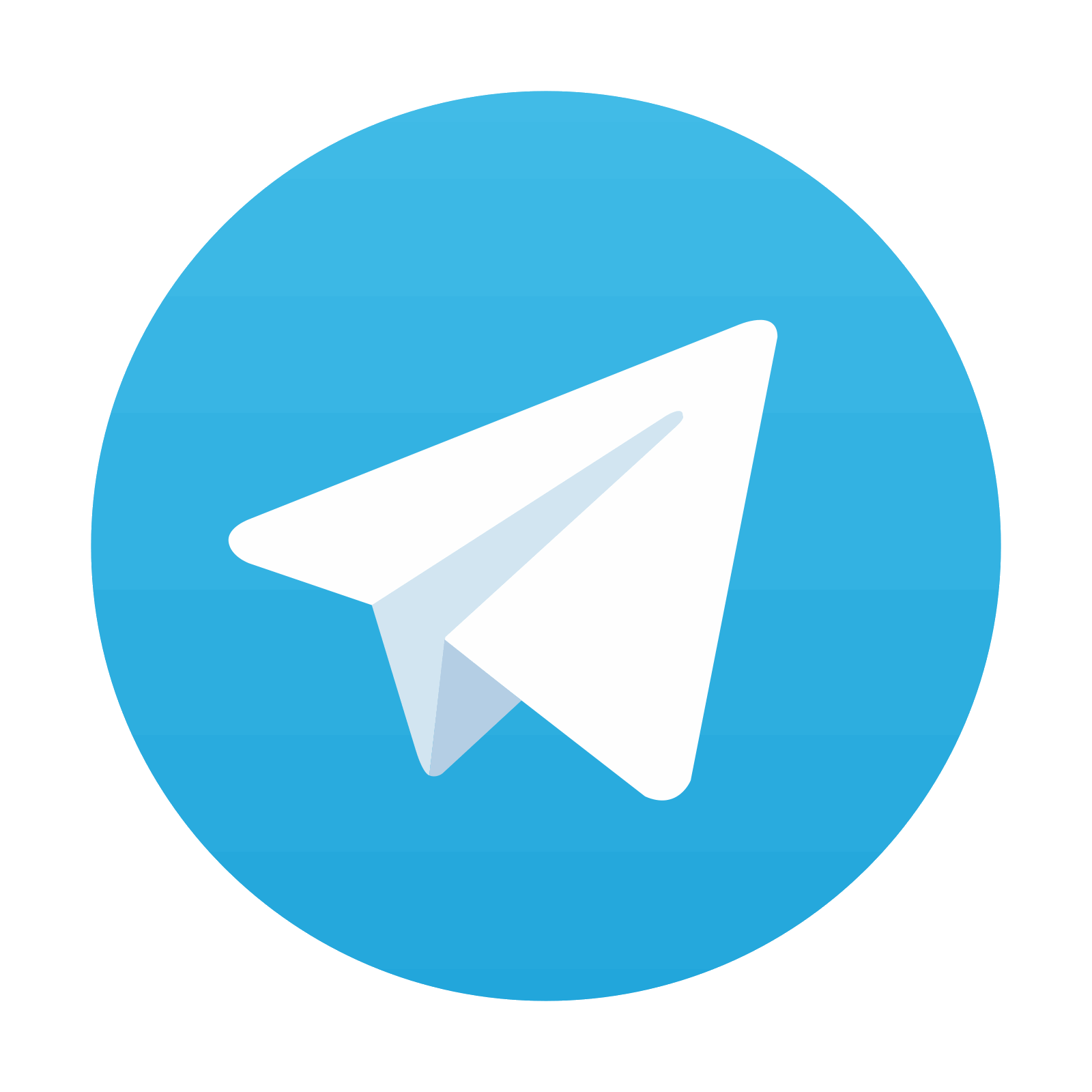
Stay updated, free articles. Join our Telegram channel
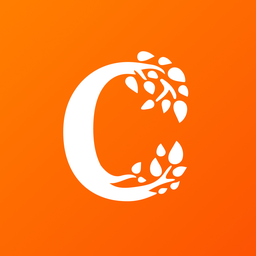
Full access? Get Clinical Tree
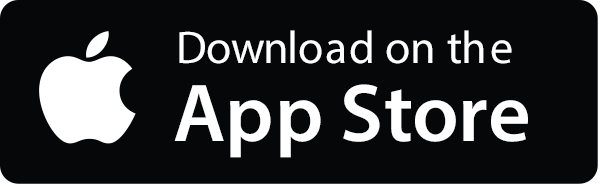
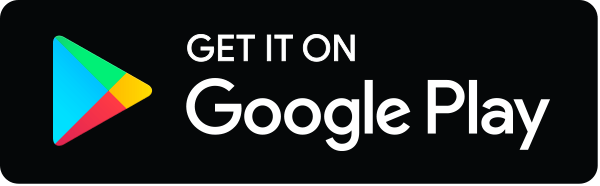
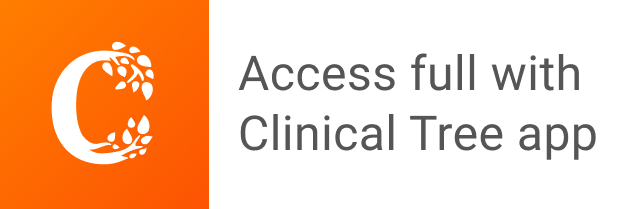