Fig. 35.1
Animal models of heart failure. (a) Sham (b) Pressure overloaded model after suprarenal ligation (c) Volume overloaded model after mitral regurgitation (d) Mixed load remodeling model after myocardial infarction
Rat Pressure Overload Models
Aortic Banding
Models involving an abnormal pressure load have been most useful in the study of the pathogenesis of hypertrophy, subcellular failure, and vascular changes. There are numerous surgical methods to induce pressure overload in rats, but the ascending aortic banding is one of the more widely used surgical models, in which a stricture is placed around the ascending aorta of weanling rats [7, 8]. Compared with ascending aortic banding, abdominal aortic banding model is more physiologic model like human clinical settings. Abdominal aortic banding is also a well-established model for inducing hypertrophy due to pressure overload in animals and it has been used extensively for many decades [9, 10]. The abdominal aorta is exposed above the left renal artery and a silk thread is passed under it. A 23 cannula is placed longitudinally on the aorta and both aorta and cannula is tied. The cannula is then removed, leaving an aortic lumen determined by the diameter of the cannula (Fig. 35.2). The skin is closed by clipping and covered with tar spray. Kim and colleagues [11] have used this model extensively to explore the new phosphodiesterase 5 inhibitors (PDE 5 inhibitors), udenafil therapy to prevent heart failure and decreased inflammatory markers in pressure overloaded failing heart. They showed that udenafil-attenuated myocardial fibrosis and apoptosis. Udenafil also decreased myocardial matrix metalloproteinase-9 expression and augmented serum interleukin-10 concentration. Long-term udenafil use prevented cardiac remodeling and improved exercise capacity and survival in rats exposed to pressure-overload cardiac hypertrophy.


Fig. 35.2
The procedure of pressure overload model; suprarenal ligation
Rat Volume Overload Models
Aortocaval Fistula
Much less is known about signal systems for volume overload than those for pressure loads because there are fewer volume overload models. It is of interest that, as opposed to LV pressure overload, LV volume overload is associated with a decrease in interstitial collagen surrounding cardiomyocytes [12, 13]. The pure volume overload of aortocaval fistula (ACF) in the rat causes a LV stretch stimulus without an increase in LV pressure due to the arterial-venous shunt. As in the human with a pure volume overload, this results in an adverse LV eccentric remodeling involving increases in LV end-diastolic pressure and LV end-diastolic dimension to wall thickness ratio over a period of time. Heart failure is induced in this model by surgical creation of an arteriovenous (AV) fistula between the abdominal aorta and inferior vena cava, distal to the origin of the renal arteries [14, 15].
Mitral Regurgitation Model in Rats
Mitral regurgitation (MR) is the most common type of valvular heart disease that induces LV volume overload. In their pioneering work, Pu et al. [16] developed an MR rat model for the first time. However, their report lacked details about the animal model, LV remodeling, exercise capacity, and histological findings that are critically important for future research. Kim et al. [17] established a reliable small animal model of chronic MR using rats and verified the pathophysiological features of this model using serial echocardiography. After anesthesia, an intracardiac echocardiographic catheter is inserted into the esophagus for transesophageal echocardiography. A lateral thoracotomy is performed, and a needle is inserted into the LV via apical puncture and is advanced toward the mitral valve to create a hole in the leaflet and induce MR under the guidance of transesophageal echocardiography. Using this model, they evaluated that sildenafil attenuated LV remodeling and prevents exercise intolerance in a rat model of chronic MR likely due to the antiapoptotic, anti-inflammatory effects of sildenafil. They used transcriptional profiling of cardiac apical tissues which revealed that gene sets related to inflammatory response, DNA damage response, cell cycle checkpoint, and cellular signaling pathways were significantly enriched by genes with reciprocal changes in sildenafil treatment group (Fig. 35.3).


Fig. 35.3
Mitral regurgitation causes progressive left ventricular dilatation, wall thinning, and cardiomyocyte elongation, which recapitulates eccentric remodeling with inflammation cell infiltration, extracellular matrix degradation and activation of DNA damage pathway. The anti- inflammatory and antiapoptotic effects of sildenafil seemed to play a key role in preventing LV remodeling
Rat Ischemic Injury/Myocardial Infarction Model (Mixed Load Ventricular Remodeling Model)
Various methods have been applied to induce MI and/or ischemia in animals via occlusions of coronary arteries. Left ventricular MI in rats has been established by Pfeffer et al. [18] In brief, after anesthesia, orotracheal intubation and thoracotomy, the heart is rapidly exteriorized and the LCA is ligated in the proximal segment using a thin thread. The occlusion of the artery can be recognized by blanching of the tissue distal to the ligation. Rats with infarctions greater than 46 % develop congestive HF after 21 days with elevated filling pressures, reduced cardiac output, and a minimal capacity to respond to pre- and after-load stress. The degree of impairment of LV function is directly related to the extent of myocardial loss. In comparison to the permanent occlusion model, the reperfusion MI model leads to a higher infiltration of inflammatory cells, attenuated fibrotic remodeling and enhanced neovascularization in the area of infarction [19].
Methodological Considerations
HF animal models must be carefully characterized to ensure that they have the critical features that have been described above. The level of characterization will ultimately be determined by the study design and the available equipment and resources. Several additional points are worth considering as work continues in animal models: (1) Our first concern was that heart size and gender have been neglected in some previous experiments. In our pilot study, we found that rats showed rapid growth of the heart between 8 and 10 weeks of age (LVESD, 3.40 ± 0.15 mm vs. 4.02 ± 0.27 mm; LVEDD, 6.41 ± 0.34 mm vs. 7.31 ± 0.34 mm for 8 vs. 10 weeks of age, p < 0.01); thus, this period was not optimal for evaluating LV remodeling (Fig. 35.4). (2) When considering new treatment approaches, experiments that determine whether treatment reverses ventricular remodeling are more relevant than animal studies of prevention of heart failure; (3) Complete hemodynamic assessment of the animals used is essential, including assessment of both static and dynamic parameters as well as structural remodeling in determining the magnitude of these parameters. Assessment of ventricular cardiac performance is also of importance; (4) Assessment of the functional capacity of both diseased and treated animals to determine whether improvement in hemodynamics and cardiac function afforded by pharmacological intervention is clinically significant; (5) Longer-term studies on the effects of treatment must be performed with effects on survival and toxicity being included. We will mention shortly the approaches that have been used routinely to reliably characterize small-animal heart failure models are outlined below.


Fig. 35.4
In Kim et al. pilot study, they found that rats showed rapid growth of the heart between 8 and 10 weeks of age (LVESD, 3.40 ± 0.15 mm vs. 4.02 ± 0.27 mm; LVEDD, 6.41 ± 0.34 mm vs. 7.31 ± 0.34 mm for 8 vs. 10 weeks of age, p < 0.01); thus, this period was not optimal for evaluating LV remodeling
In Vivo Cardiac Function
Transthoracic echocardiography can be used to evaluate cardiac function in anesthetized or conscious rats [17, 20–22]. LV septal and posterior wall thickness (SWT and PWT, respectively) and the LV end diastolic/systolic dimension (EDD/ESD, respectively) can be measured using M-mode echocardiography at the papillary muscle level. The LV ejection fraction (EF) and LV mass can be estimated by established formulas [23]. Transthoracic 2-dimensional guided M-mode echocardiography and pulsed-wave Doppler wave can be performed by using a variety of commercially available echocardiograph machines (Fig. 35.5). Serial measurements should be performed to evaluate structural and functional progression of heart failure or to evaluate the efficacy of therapeutics. Recent miniaturization and refinement of imaging technology for rats such as high-field-strength MRI machines (9.4 T) have facilitated noninvasive characterization of the pulmonary and systemic circulation in rodents [24, 25]. However, cardiac MRI of small animal model needs lots of time to get the image, so anesthesia time could affect the result of study.


Fig. 35.5
Echocardiography (a) 2D & M-mode. Color Doppler. (b) Spectral Doppler. Mitral inflow. (c) Tissue Doppler. Mitral annulus
In Vivo Measurements of Exercise Capacity
Measurements of exercise capacity is very important parameters in patients with heart failure. Exercise capacity of rats can be measured by several methods. Kim et al. used the Rotal Rod Treadmill [17]. The rats ran on a knurled drum as the drum rotated to avoid falling off. Animals were trained two times before the test to adjust the treadmill. Treadmill speed was gradually increased from 3 revolutions per minute (rpm) to 15 rpm every 1 minute, and maximum exercise time was recorded. Guazzi et al. [26] measure maximal exercise capacity with a conventional motor-driven treadmill. They had the 2-week acclimatization period. During the first week of acclimatization, this consisted of 3 sessions of slow walking on the treadmill for 10 to 15 minutes each. During the second week of acclimatization, rats underwent 3 maximal exercise capacity tests, with the final 2 averaged. If there is special chamber, maximal VO2 can be measured. The animals were placed on a treadmill and enclosed in an airtight metabolic chamber. This chamber was adapted for the determination of O2 uptake (VO2) by using an open-circuit method [27].
In Vivo Measurements of Loading Cardiac Conditions
Thanks to advances in microcatheter technology, ventricular pressure-volume relationships has been successfully translated to small animal models, including rats, allowing for detailed characterization of cardiovascular function. This method is unique for providing measures of ventricular performance that are more specific to the heart and less affected by vascular loading conditions. Pacher et al. showed the protocol in depth and detail in Nature Protocols [4]. Despite its invasiveness, this sophisticated methodology has great potential for characterizing cardiac function in various rodent- models of cardiovascular disease.
Conclusions
There is not yet a perfect preclinical model that accurately reproduces all the clinical pathological features of human heart failure. However, it is also clear that animal models have provided, and will continue to provide, valuable insight into the numerous pathways that contribute to the development and maintenance of heart failure. These models will allow us to investigate important interactions between the various triggers, which have been implicated in heart failure, their impact on signaling pathways, and their temporal evolution into the structural and functional abnormalities, which characterize the myocardial dysfunction process. Use of both classic and newly developed animal models will allow us to continue to rigorously test new hypotheses regarding pathogenesis and evaluate the ability of newly developed agents for prevention and reversal of established disease. Careful and rigorous clinical trials will be required to establish safety and efficacy of any heart failure therapy or mechanism in human patients.
Pathophysiology
Heart failure is described by a complex pathophysiology that includes more than just the cardiovascular system. Heart failure is often thought of in the context of the “neurohormonal hypothesis.” This hypothesis states that that neurohormonal influences involving imbalance of sympathetic and parasympathetic tone as well as disruption of the renin-angiotensin-aldosterone system contribute to heart failure [28]. Additionally, deleterious systemic inflammation involving various cytokines has been observed to further the progression of chronic heart failure, as postulated by the “cytokine hypothesis” [29]. Although the relationship between inflammatory cytokines and the neuroendocrine system has not been fully elucidated, studies show that the two systems influence each other to advance the status of heart failure [30]. Further, recent studies have shown that the two systems may interact with each other in the pathogenesis of this disorder [31]. This section will discuss the role of inflammation and neurohormonal influences in the pathophysiology of heart failure, as well as other aspects of heart failure syndrome such as cachexia, endothelial dysfunction, and reduced skeletal muscle blood flow.
Cytokines in Heart Failure
Elevated circulating levels of pro-inflammatory cytokines are seen with heart failure. The most important cytokines are TNF-α, IL-6, and IL-1. A number of chemokines, including macrophage chemoattractant protein (MCP)-1, IL-8, and macrophage inflammatory protein (MIP)-1α are also associated with heart failure; these levels of chemokines increase with heart failure progression. Increased expression of inflammatory cytokines and chemokines has been demonstrated by Damas et al. at both the protein and mRNA levels in a study of patients with heart failure; particularly high levels of these pro-inflammatory cytokines and chemokines were found in the coronary circulation [32]. The pro-inflammatory effects seem to be unopposed due to the absence of anti-inflammatory cytokines such as IL-10. The result of this imbalance leads to a systemic inflammatory effect [33]. The causes of inflammation in heart failure are manifold. The initial inflammation in early stages of myocardial infarction activates an immune response that can potentially explain some of the pro-inflammatory signals in heart failure. Moreover, mechanical overload and shear stress cause cytokine expression in various cell types including the endothelium, leukocytes, and even the cardiomyocytes themselves, suggesting these are native molecules made not just by the immune system but within the heart tissue itself. The consequential effects of these pro-inflammatory cytokines include release of radical nitric oxide, oxidative stress, and apoptosis among many other outcomes. Here, we describe the individual roles of TNF-α, IL-6, IL-1, TLRs and MMPs for their contribution to the pro-inflammatory state during heart failure and their intrinsic connection to neurohormonal activation.
Tumor Necrosis Factor Alpha (TNF-α)
TNF-α is a cytokine released by myocardial cells in response to damage and thought to be the one of most important mediators of HF progression. TNF-α exerts its effects via TNF-α receptors (TNFR), which are ubiquitously expressed. Circulating levels in TNF-α levels are elevated in patients with CHF and increases as heart failure worsens [34], demonstrating a positive association between TNF-α and the progression of HF. TNFR then modulates myocardial function through two ways: (1) instantaneous activation of the sphingomyelinase signaling pathway that occurs within minutes of ligand interaction and (2) a slower, delayed response that involves the inhibition of β-adrenergic by nitric oxide [35]. TNFR-1, one subtype of the TNF receptor, is more abundantly expressed and mediates the majority of TNF-α’s deleterious effects such as remodeling, hypertrophy, nuclear factor-kappa-B production, and apoptosis. TNF-α mediates its apoptotic effects through its effect on the death domain within the cytoplasmic portion of the TNF receptor-1, and secondarily activating NF-kB. Additionally, cytokines exert a cytotoxic effect on cardiomyocytes, leading to necrosis [36]. NF-kB effects and necrosis, together, further compromise the composition and function of the heart. TNFR-2, which is less commonly expressed, actually provides a protective effect for the heart. A study with patients with myocardial infarction by Valgimigli et al. demonstrated that TNFR-1 was the most powerful independent risk factor for HF and death [37]. Yet despite the contradictory effects of the TNFR-1 and TNFR-2, Hamid et al. implicated that both receptors are necessary in precipitating the pathophysiological consequences of TNF-α [38], including left ventricular dysfunction and remodeling, cardiac myocyte apoptosis, development of anorexia and cachexia, reduced skeletal muscle blood flow, and endothelial dysfunction, and other effects.
Interleukin 6
Increased concentrations of circulatory IL-6 are also strongly associated with advancement of HF. Moreover, in hospitalized patients with CAD, IL-6 levels were significantly associated with all-cause and cardiovascular mortality [39]. IL-6 type cytokines mediate their signals through the ubiquitously expressed glycoprotein (gp) 130 receptor subunit, which is central to the inflammatory effects observed in HF.
Short-term activation of IL-6R confers protective effects during ischemia partly through its classical, membrane-bound signaling. However, constitutive, persistent activation of gp130 present during heart failure states contributes to cardiac hypertrophy, myocardial dysfunction, and muscle wasting via IL-6 trans-signaling pathway [40]. Nevertheless, it has also been shown in genetic models of IL-6 that IL-6 is not necessary for the pathogenesis of heart failure. Fuchs et al. showed that IL-6 knockout mice did not have differences in LV dysfunction and hypertrophy after MI compared to regular mice. They postulated that there were redundancies between this system and the JAK/STAT pathways such that there were compensatory mechanisms through alternative receptors [41].
Interleukin 1
IL-1 works synergistically with TNF-α to depress myocardial contractility in a dose-dependent manner. IL-1, similar to TNF-α, is a potent inducer of myocardial apoptosis and hypertrophy in vitro [42, 43]. Further, it is involved in arrhythmogenesis, leading to exacerbation of HF [44]. Recent studies have suggested that TNF and IL-1 may have negative inotropic effect on the heart indirectly through activation and release of IL-8 [45, 46]. Preclinical studies suggest that IL-1 blockade has a positive effect on the failing heart, and clinical trials are under way to investigate the role of IL-1 blockade in improving symptomatic outcomes in patients with HF [47].
Toll-like Receptors
Toll-like receptors (TLRs) are also speculated to be an important link to inflammation in HF. TLRs are pattern-recognition receptors that are primarily involved in initiating the innate immune responses in the presence of conserved microbial molecules. Studies show that in HF that arises after acute myocardial infarction, TLRs are activated on monocytes. For example, in a study of patients with acute myocardial infarction by Satoh et al., TLR4 levels on monocytes positively correlated with IL-6 and TNF-α levels [48], indicating that activation of TLR4 through a myocytic inflammation reaction is associated with HF post-MI. This evidence is strengthened by inflammatory cytokines’ upregulatory effect on TLR2 and TLR4 of vascular endothelial cells, which is likely to contribute to endothelial cell-related inflammation in HF. Additionally, enhanced effects of TLR2 and TLR4 are seen in injured myocardium, which subsequently recruit cells of innate immunity that may contribute to the myocardial inflammation in HF progression. The link between TLR and inflammation can be explained by TLRs’ capacity to respond to not only microbes, but also to molecules released from injured cells, such as fibronectin and heat shock protein 60 [49].
Matrix Metalloproteases
Inflammatory cytokines, particularly TNF-α, are important regulators of myocardial activity of a proteolytic system called matrix metalloproteinases (MMPs) and their inhibitors, tissue inhibitors of metalloproteinases (TIMPS). During left ventricular remodeling and heart failure, a fine balance exists between matrix metalloproteinases, which are responsible for digesting extracellular matrix, and TIMPs, their inhibitors. It has been shown that through the different stages after MI leading to heart failure that there are important spatial and temporal changes that correspond to functional outcomes. TNF-α, along with numerous other cytokines, is overexpressed by cardiomyocytes and causes the induction of MMP activity. The increased MMP proteolytic activity relative to TIMP activity results in disturbance of fibrillar collagen and ultimately fosters ventricular dilation. Thus, TNF-α is seen to influence left ventricular (LV) remodeling, a marked characteristic of heart failure, through the induction of MMP species. Interestingly, this phenomenon is short-lived. As time progresses, the MMP activity appears to decrease in response to TNF-α and TIMP increases, leading to increased collagen content and cardiac fibrosis [50].
Neurohormonal Activation
It has been well documented that heart failure is characterized by neurohormonal activation, in addition to inflammatory events. Neurohormonal activation is a process that involves renin-angiotensin-aldosterone system (RAAS) and the adrenergic system. Both systems are activated almost simultaneously upon damage to the myocardium, resulting in an increase of norepinephrine, angiotensin II, endothelin, TNF-α and aldosterone [51], in order to improve the mechanical state of the heart.
Angiotensin II (Ang II), a major factor in RAAS, is a robust vasoconstrictor of the renal efferent arterioles and systemic circulation that is produced during states of low cardiac output. Ang II also stimulates release of norepinephrine, from sympathetic nerve terminals and induces the release of aldosterone and AVP, both of which increase circulating blood volume through retention of sodium and/or water. Low cardiac output also activates SNS, which promotes vasoconstriction of peripheral vessels. While these effects can be beneficial in restoring cardiac output in a functional heart, persistent activation of both systems can have catastrophic effects on cardiac function, particularly in an already compromised heart. A mechanism through which this may occur is through the combined vasopressor effects by RAAS and SNS, which induce a large increase in left ventricular afterload. This, in turn, results in elevated myocardial demand, pulmonary capillary wedge pressure, and left ventricular end-diastolic pressure, all of which contribute to progression of HF. Furthermore, increased blood volume by aldosterone and AVP results in increased intracardiac pressure, pulmonary congestion, and edema [52]. Excessive sympathetic activity is also directly correlated to cardiac myocyte apoptosis, hypertrophy, and focal myocardial necrosis [53]. Additionally, Ang II also directly induces cardiac myocyte necrosis and modifies the myocardial matrix structure [54], latter by promoting reactive perivascular and interstitial fibrosis. It is not surprising, then, that the degree of neurohormonal activation is correlated with severity of HF. Francis et al. studied neurohormonal activation among patients with left ventricular dysfunction with and without congestive heart failure, which demonstrated plasma norepinephrine, plasma AVP, and plasma renin increase as heart failure progresses [55]. Further, the extent of neurohormonal activation is correlated with prognosis of HF. For example, the Cooperative North Scandinavian Enalapril Survival Study demonstrated that levels of angiotensin, ANP (atrial natriuretic peptide), norepinephrine, and epinephrine were significantly higher in patients who died than those who survived in a study of patients with severe heart failure randomized to treatment with enalapril or placebo [56]. The association between neurohormonal activation and long-term prognosis of HF is well evidenced by studies that demonstrate improvement in cardiac function and reduced mortality after administration of inhibition of neurohormonal activation in patients with HF. In the Metoprolol CR/XL Randomised Intervention Trial in-Congestive Heart Failure (MERIT-HF) study, metrolol, a beta blocker, given once daily in addition to optimum standard therapy improved survival of patients with chronic heart failure [57]. β-blockade is thought to suppress many deleterious hemodynamic and metabolic effects of SNS and at least partially interfere with the RAAS in patients with HF [58]. Analogously, ACE inhibitors and angiotensin II receptor blockers suppress neurohormonal activation by inhibiting the effects of angiotensin.
It is important to note that inflammatory and neurohormonal activation in HF progression are not independent processes. Studies have demonstrated that angiotensin II has significant proinflammatory effects in the vascular wall, inducing activation of leukocytes and synthesis of adhesion molecules, ROS, and inflammatory cytokines, which facilitate in endothelial dysfunction and vascular inflammation [59]. Further, activation of the SNS primarily suppresses the activity of the innate immune system while it modulate the cells of the acquired immune system [60]. Gurantz et al. demonstrated that TNF-α and IL-1 are directly related to increasing angiotensin II receptor density in the myocardium [30]. Further interplay between these two systems is further detailed in pre-clinical animal studies, suggesting a complex process that needs to be better understood.
Clinical Disease
Heart failure can either be acute or chronic, and is characterized by inability of the ventricle to fill with or eject blood. The inability leads to decreased cardiac output that can no longer meet the body’s nutritional demands. Moreover, blood backs up and becomes congested in the lungs, liver, and systemic circulation. This leads to a variety of symptoms: dyspnea, orthopnea, paroxysmal or intermittent nocturnal dyspnea, fatigue, cyanosis, and edema. As described in previous sections, activation of neurohormonal and inflammatory systems occurs both in response to heart failure in the body’s attempt to restore blood pressure and blood volume and thus, cardiac output.
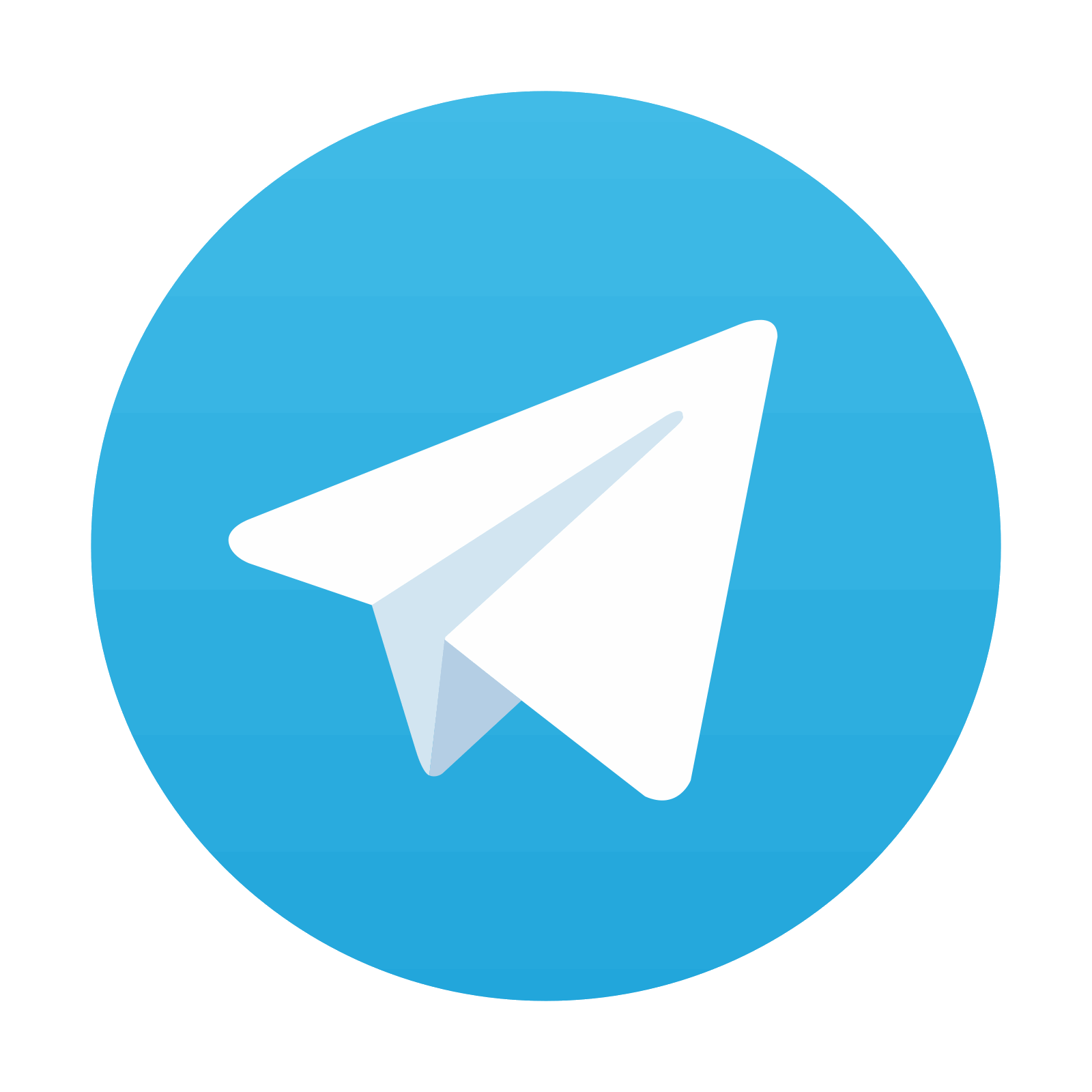
Stay updated, free articles. Join our Telegram channel
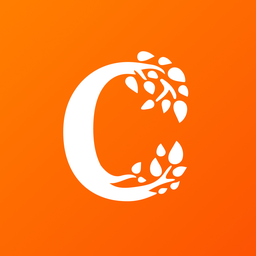
Full access? Get Clinical Tree
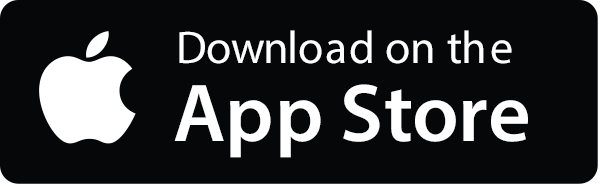
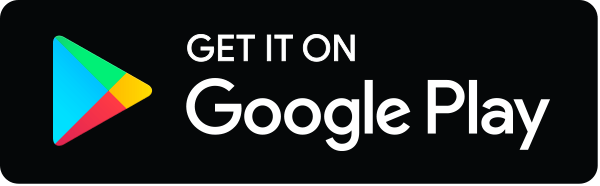