Significant studies
Combination of cardiovascular telemetry data collection with calorimetry studies allowed to determine metabolic rates in rodents [46]
Combination of telemetric blood pressure measurement with flowprobes to simultaneously monitor pressure and cardiac output, or pressure and renal flow simultaneously, though the flow measurement still requires external leads and tether connections [47]
Miniaturization of transmitters capable of being use in cases in which space in the abdominal cavity is particularly limited such as during pregnancy and small mice [48]
Studies that involve collecting respiratory data have generally required restraining the animals and placing a pneumotach on their face to collect respiratory data. This method can be stressful for the animal and thus limits data collection to short periods of time. Alternatively, with external telemetry and respiratory inductive plethysmography (RIP), the animal is unrestrained and is therefore presumably less stressed, allowing data to be collected continuously [49]
A new transmitter enables simultaneous collection up to four parameters, such as body temperature, activity, blood pressure, ECG, and other biopotentials in small animal models, besides information about battery life, animal identification, and calibration information. This contrasts with previous transmitters that could measure either blood pressure or ECG parameters. This new device eliminates the need for separate animal groups for assorted measurements and allows for a more complete cardiovascular assessment [50, 51]. http://www.jove.com/video/3260/implantation-radiotelemetry-transmitters-yielding-data-on-ecg-heart
Simultaneously measurement of pulmonary and systemic blood pressure and the electrocardiogram in rats using dual blood-pressure telemetry transmitter. The transmitter was implanted in normotensive and monocrotaline-induced pulmonary hypertensive Wistar rats, with sensing catheters placed in the pulmonary artery and descending aorta. Biopotential electrodes were positioned to record an apex-based lead II electrocardiogram [52]
Merging cardiovascular telemetry data collection with metabolic treadmill studies in rodents to determine VO2/CO2 consumption combined with telemetry-derived parameters
Implantable blood pressure telemetry combined with cutting-edge echocardiography to combine arterial pressure measurements with cardiac output, assessing pressure-diameter curves, amongst other parameters
Synchronizing data collected from a respiratory chamber and an implantable telemetry (intra-pleural pressure telemetry signal) obtaining more complex respiratory data
Telemetry (TEL) minimizes interanimal variability and the number of animals per protocol as the same animal can be its own internal control and several tests can be repeated in the same animals. Thus this technology represents the most humane method of assessing physiological parameters in laboratory animals, contributing to animal welfare (reduction and refinement alternatives) and reducing overall animal research costs [1].
Telemetry transmitters are surgically implanted or externally mounted devices that sense, process and transmit physiological information via electromagnetic radio waves (3 Hz–300 GHz), from a freely moving animal to a located receiver. Traditional implantable TEL devices range in size from approximately 1–35 cm3, are biocompatible, typically have permanently affixed leads, and can transmit signals up to 5 m. External devices are approximately 150–200 cm3; the larger size accommodates a bigger battery and the electronics required to transmit many signals from a single animal in a room that may contain up to 36 animals. Transmitters for blood pressure measurements utilize fluid filled catheter technology for arterial placement, containing a biocompatible gel that transduces blood pressure fluctuations back to the transmitter. Once the signal is received, it is transferred to a data acquisition computer for processing. Data collection is initiated by touching the animal with a magnet, switching on the transmitter. The acquisition program collects data signals sent to the computer from the converters and receivers. This program can either collect data for a specific period of time at regular intervals or sample continuously and save the data on the computer’s hard drive. As the range and the quality of the emitted signal depends strongly on the material composition of the cage and surrounding equipment, it is suggested that the receiver plate is placed as close to the animal as possible. After the data have been gathered and stored, they can be plotted, listed and analysed for a variety of different parameters using the analysis program (Fig. 21.1).
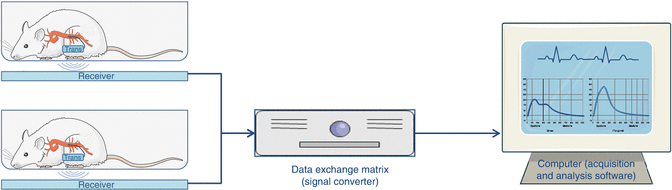
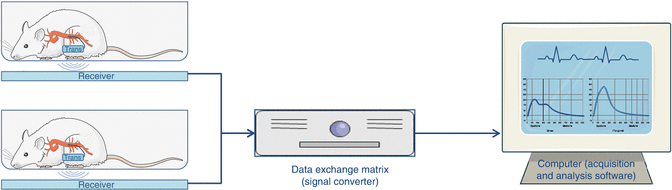
Fig. 21.1
Diagram of a radiotelemetry system. A rat implanted with a transmitter on its abdominal cavity, capable of monitoring blood pressure via the abdominal aorta. The transmitter emits a radio signal that is detected by a receiver beneath the cage. This signal is sent to a data exchange matrix (signal converter) and afterwards to the computer where the acquisition and analysis software displays the results (These images were created using Servier Medical Art and licensed under a Creative Commons Attribution 3.0 Unported License)
The animal surgical protocol for a blood pressure transmitter implantation in the abdominal aorta includes fasting the animal 12 h before surgery and preparing it for aseptic surgery using sterile instruments. The surgery itself comprises an aseptic laparotomy to expose and dissect the abdominal aorta approximately 15 mm below the left renal artery and vein, followed by a temporarily aortic occlusion for less than 1 min using a ligature above and other bellow the dissected aorta. Next, a small puncture is performed using a 25 or 26 gauge needle 5 mm below the left renal vein and the catheter tip inserted through the puncture into the aorta. After hemostatic closure of the aortic puncture with medical glue, the occluding ligatures are released. Finally, the telemetric transmitter is placed within the abdominal cavity or subcutaneously. For ECG and EEG measurements the positive and negative electrodes are placed subcutaneously. For blood pressure measurements one or more fluid-filled catheters are placed into the appropriate blood vessel (usually the carotid artery, thoracic aorta, femoral artery, pulmonary artery, or abdominal aorta). For measurements of respiratory pressure changes, a fluid-filled catheter is introduced into the pleural cavity. Once the equipment is placed and checked for proper function, all incisions are closed and the animals are permitted 1–2 weeks recovery from surgery with appropriate analgesics before studies are conducted. Potential adverse effects resulting from this procedure may include anaesthetic related respiratory distress, infection of the subcutaneous pocket, abdominal cavity or catheter insertion site, dehiscence of the surgical site, seroma formation around the transmitter, hind limb paresis or paralysis related to ischemia or nerve damage and hemorrhage due to leaking of the vessel around the catheter insertion site.
Advantages of TEL include: (1) Enabling long-term recordings; (2) Monitoring conscious rats in natural environments; (3) Reducing handling-induced stress artefacts; (4) Allowing to use animals sequentially as their own controls or in multiple studies (number reduction); (5) Decreasing animal maintenance costs; (6) Allowing to understand the complex physiological interactions between cardiovascular, respiratory and central nervous system; (7) Providing objective biological data on animal wellbeing to help implement humane endpoints (refinement). However, this methodology presents some disadvantages such as: (1) Using external or abdominal devices and mountings, which can cause discomfort and distress to the animal; (2) Requiring the animals to undergo surgery with anaesthesia to implant the transmitters; (3) Acquisition of expensive equipment and costly charging of batteries that is usually completely recovered through animal use reduction; (4) Continuous sampling generates large amounts of data, which can lead to analysis problems and (5) Requiring appropriate surgical training to implant a TELE device in an animal. Measures that can be taken to refine surgical implantation procedures include ensuring that the animals have adequate recovery time, using appropriate anaesthetics (inhalatory anaesthetics such as sevoflurane or isoflurane are recommended for telemetry procedures), using appropriate analgesia, and proper aseptic technique [2].
In conclusion, TELE systems are versatile and enable valuable physiological information to be collected from animals for a variety of different research needs. Combining telemetry with other methodologies has boosted and significantly improved in vivo assessment of cardiovascular function, providing important tools to maximise the information gained and thus understand the pathophysiology of cardiac disease in animal models.
21.2.2 Echocardiography
Echocardiographic evaluation is a powerful tool for non-invasive evaluation of both cardiovascular structure and function. It is a relatively quick and versatile method that allows serial assessment. Considerable developments in technology and equipment in the last decade have made possible a thorough evaluation of cardiac structure and function even in mice. New transducer with small footprints, capable of both high frame-rates and improved near-field imaging are now available which enable fabulous views with outstanding temporal resolution even in small rodents [3]. This equipment however is extremely expensive and many cardiovascular function parameters can still be evaluated with more affordable echocardiography machines. Moreover, we must point out that ECHO is not an automated procedure. It is highly operator dependent and relies on the proper acquisition and interpretation of results by an examiner who is familiar with the principles, capabilities, and limitations of ultrasound imaging rather than on the equipment itself [4]. Because of the important operator dependency in experimental settings whenever possible the examiner should be blinded.
21.2.2.1 Principles of Echocardiography
It is beyond the scope of this chapter to exhaustively revise the principles of ultrasound imaging. Briefly, image is formed by tissue reflection of sound waves above the range of human hearing generated by piezoelectric crystals. Echocardiography probes or transducers interconvert electrical energy in ultrasound upon emission and reception. The piezoelectric crystals deform when exposed to a strong electrical field and emit ultrasound. Then again they also deform mechanically when they receive the echoes of emitted ultrasound and generate a voltage. The oscillation upon electrical stimulation has a specific frequency that varies inversely with crystal thickness. Crystals in transducers are covered by damping materials and acoustic lenses. The ultrasound waves are sent out in a cylindrical pattern up to a certain distance, the near-field, and then diverge conically in the far-field. The lateral edges of the ultrasound beam are not abrupt. Beam width defines lateral space resolution which is the minimum distance at which two separate reflectors will be perceived as such by generating separate echoes. Beam width decreases with increasing frequencies. The more recent ECHO machines use a method called harmonic imaging to further reduce beam width and artefactual images from multiple reverberations by filtering the received signals. Strong reflectors such as metal are usually perceived further to the edge of the beam and thus appear larger, an artefact called blooming. Despite all adjustments diffraction phenomenon still generate oblique weaker secondary beams known as side lobes that are responsible for side-lobe artefacts. To increase the width and tissue depth of the image crystals are usually grouped and closely spaced in the probe. Additionally, they can be rotated in a fan-like fashion by mechanical sector scanners. Moreover pulses of ultrasound waves can lag between each of the crystals (phased array) so that during sector scanning the wave fronts from the crystals form an arc which constitutes a slice or wedge of the scanned object. Phased arrays increase the ability to scan wide fields of tissue in deeper planes. If the crystals emit simultaneously a (linear phase) beam is formed perpendicular to the probe and a rectangular tissue section is imaged. Linear arrays are informative on a wide region of tissue close to the transducer and are usually used for superficial imaging.
Ultrasound waves change pressure within the medium which they traverse periodically over time and space with both a set frequency which is the reciprocal of their period, or time between successive pressure peaks and a set wavelength which is the distance between pressure peaks of an ultrasound wave. The wavelength (λ) relates to the frequency (f) depending on the speed of propagation within the medium (c):
The speed of propagation varies proportionally to materials or tissues stiffness and therefore it is higher in solids and lower in gases. Wavelength is the determinant of space resolution. Higher frequencies therefore provide better resolution. Additionally waves also have an amplitude that relates both to the changes in pressure within the tissue they traverse and to the power and voltage of emission and reading, respectively, in the ECHO machine. For most purposes, including 2D ECHO ultrasound, waves are released in pulses interspersed by long periods of silence for returning echo analysis. Therefore ultrasound waves also have pulse duration, pulse repetition frequency and a duty cycle or the percentage of time that the pulse of ultrasound is on. Duty cycle and wave amplitude are the determinants of power output.

The image is formed upon reception of the echoes. In 2D or brightness mode (B-mode) echocardiography a selected group of crystals emit ultrasound as defined by the sector scan, with a certain pulse repetition frequency. When waves are emitted they perform a round-trip travel from probe to tissue and back. For an accurate analysis there must only be one pulse of ultrasound in the tissue in every period of analysis. This means that higher pulse repetition frequencies do not allow time for tissue penetration. To increase temporal resolution (frame rate) in order to assess fast-moving objects, both sector scan and sector depth should be reduced to enable high pulse repetition frequencies. The highest frame rate is achieved by reducing sector scan to a single line as in motion-mode (M-mode). In this case visual representation is a plotting of brightness along that line as a function of time. This view allows the best temporal resolution when measuring distances between rapidly moving objects. Nonetheless, storage in video format has a lower rate; for higher rate storage digital storage media must be used. Echo signals are converted digitally to pixels in a grey scale. The dynamic range is the ratio between the strongest and weakest echo signals that can be distinguished in this scale. The higher resolution component in ECHO machines is range resolution, or axial resolution along the beam, which depends mostly on frequency. For better range or axial resolution the surfaces of interest should be as perpendicular as possible to the beam. When measuring the axial distances between objects in M-mode or 2D-echocardiography the aspect of resolution should also be taken in account, contrasting interfaces between surfaces will appear as a trail with a leading and a trailing edge, measurements should be made between the leading edges to minimize errors in estimation and by convention (leading edge-to-leading-edge) [5].
Ultrasound images are formed mostly by wave reflections from tissues which define the major anatomic features, and by tissue scattering which defines the texture of tissues. Reflections occur mainly due to sudden differences in acoustic impedance which is mainly dependent on density. When these differences are too pronounced they prevent imaging in deeper planes, this is the case of the tissue-air and tissue-bone interfaces in the lung and rib-cage. Reflections are maximal when the interface is perpendicular to the direction of ultrasound and is almost absent when it is parallel. This is why endocardial and epicardial borders and poorly viewed in the 3 and 9 o’clock positions in a cross-sectional short-axis left ventricular view. Scattering is more important in non-homogeneous tissues such as the myocardium and less in blood, which appears echo-lucent. During deeper tissue penetration ultrasound waves suffer attenuation this is to say they progressively lose amplitude (power) because the ultrasound energy is converted in heat within tissues and part of it is reflected or scattered. To compensate for this loss most ECHO machines allow automatic or manual time-gain compensation that increases the intensity of images in deeper tissues.
Besides blooming and side-lobe artefacts, other typical sham images are ladder–like or comet tail appearances which result from unusually strong reflections or reverberation between two highly reflecting structures. Particular cases are the mirror image which is typical of vessel structures and consists of a duplication of a strongly reflecting interface at an equal distance but further away from the probe and the near-field clutter when highly reflecting structures are near the probe. Another common artefact is shadowing beyond an unusually reflective structure which is due to reduction in ultrasound energy.
Although we previously stated scattering was less important in blood it is the weak backscattering from blood cells that is used to perform Doppler ECHO. Blood cells are much smaller than the wavelengths of the ultrasound waves and actually have no reflection at all but only very weak scattering signals. The Doppler effect first described for light by Christian Doppler, is the wave frequency change that takes place when the emitter and reflector are in motion relative to each other. In a simplistic view, frequency increases when they approach because wave fronts are squeezed foreshortening the wavelength and increasing the frequency. When the emitted signal is combined with the received signal in the ECHO machine a difference, or Doppler shift, is computed. According to the original Doppler equation the frequency of emission (f 0) is changed to a Doppler frequency (f D) as a function of the velocity of motion between the emitter and the receptor (v), which must be accounted for twice, and the speed of propagation in the medium (c):
As an approximation since v is of a very lower magnitude compared to c, the equation is usually simplified to:
Doppler’s equation however only applies to objects that move in a straight line towards each other. For oblique movements only the vector component of velocity that falls in the line directly connecting the objects should be taken in account and the angle of movement must be corrected for (θ):
Corrections to θ <20° are usually minimal otherwise the software of the machine usually allows manual insertion of an angle for correction. Nevertheless since the flow is actually tridimensional the researcher should try to get several readings and stick with the highest value. For Doppler ECHO recordings the ultrasounds are of lower frequency and higher amplitude to increase the strength of the scattered signal and the main output is to ascertain velocity from the frequency change or shift. The Doppler shift is in the human hearing range and can be converted to sound. Alternatively using a Fourier transformation the echocardiography machine analyses the full spectrum of returned frequencies (or velocities) and their corresponding strengths and plots them as a function of time. Spectral analysis is required because scattering occurs in a pool of sampled blood and tissue and therefore frequencies/velocities vary. In every vertical line all velocities are represented and their strength is reported by a grey scale. To prevent strong low-velocity reflections from tissue a low-velocity wall-filter is usually applied. Spectral analysis and display can be done either with continuous-wave or pulsed-wave Doppler. In continuous-wave Doppler some crystals continuously emit ultrasound whereas others receive them. The spectrum received results from superimposition of all the echoes returning from all depths along the ultrasonic beam continuously and therefore higher-speed signals can be recorded unambiguously but not their precise location. In contrast to continuous-wave Doppler pulsed wave Doppler uses the same crystals as emitters and receivers in a pulse and repetition manner and can be viewed along with a 2D-ECHO image at a low rate. The researcher can select the targeted sample blood volume area along the Doppler line and then toggle to spectral Doppler view with higher temporal resolution. The spectral reading is usually more linear because velocities are more homogeneous unless an area of turbulent flow is selected. Pulsed-wave Doppler in contrast to continuous-wave Doppler, however, does not measure maximum velocities as accurately and if these are fast such as those across a stenotic or banded vessel or across the tricuspid valve in regurgitation it is unable to measure them at all. When the Doppler shift exceeds half the pulse repetition frequency, the velocity of objects falls outside the limits of display and gives rise to a false record usually indicating reversal of flow. This phenomenon is called aliasing and the limit of velocity for its occurrence is the Nyquist limit. The Nyquist limit can be increased to avoid aliasing by lowering frequency and diminishing depth. In the device the scale and baseline should also be adjusted. In rodents, some speeds can only be recorder with continuous-wave Doppler, for instance when a banding is applied to high pressure vessels such as the aorta or pulmonary artery or when assessing tricuspid regurgitation velocity. This poses a problem to the application to rodents of high-frequency linear probes commonly used for superficial structure and soft-part imaging in the clinics, because these do not have the possibility for continuous-wave Doppler. To gauge flow dynamics coding can also be done with colour superimposed on the 2D-image, with a lower temporal resolution. The scale goes from red to blue signalling velocity of approximation or departure from the probe, respectively. As for aliasing the same principles of pulsed-wave Doppler apply to colour-Doppler. Since the frequencies applied with Doppler are lower and the signal is weak it is exquisitely sensitive to electrical interference.



The same Doppler principle can be applied to tissue motion but in this case the velocities are quite lower and the signal is stronger. By adjusting scales and filters tissue Doppler images can be viewed either spectrally in pulsed-wave or in colour-mode. The velocity scale must be reduced to values near the 1–2 cm s−1, wall-filters should be removed and gain minimized. Tissue motion analysis by Doppler has many limitations; the structures in the heart are constantly moving, the myocardium contracts both longitudinally and circumferentially, and passive movements are not separable from active motion. Usually analysis is performed at the myocardium near the mitral or tricuspid annuli to assess the areas with greater excursion with pulsed-wave Doppler that is able to record maximum velocities with a better temporal resolution.
Analysis of myocardial deformation or strain and its velocity or strain rate in ECHO initially arose with tissue Doppler by comparing velocities at two distinct myocardial regions. The main advantages are the ability to better distinguish active contraction from passive motion and segmental analysis. With new software developments these can also be computed from 2D-echocardiography by tracking of speckles in the image. These are naturally occurring acoustic marks due to particular patterns of ultrasound scattering in the myocardium [6].
21.2.2.2 Tips for a Good Echocardiography Exam
Attention to details can have an important role in image optimization. Ultrasound route to the target tissue should be as clear as possible. The fur must be completely shaved and if possible a depilatory cream should be applied to minimize artefacts. The animal should be positioned in order to approximate the heart from the chest wall and increase the area not covered by the lungs which usually requires left lateral decubitus and thoracic extension. A generous ECHO gel layer or even a gel containing rubber covering the tip of the probe can minimize signal loss, increase the contact surface area between animal and probe, and serve as acoustic standoffs that gain millimetres in depth for proper tissue focus. Settings should be adjusted to obtain the optimal image or velocity recording following the previously outlined principles. Artefacts are very common in small animals several strategies can minimize them. Reducing power output and repositioning the probe usually reduces artefactual images. When doubts arise several planes and depth settings should be analysed to ascertain which images are indeed real. On screen image is usually improved simply by reducing ambient light and avoiding reflection. A simultaneous ECG acquisition is invaluable while assessing timing of phenomena along the cardiac cycle, particularly in what concerns flow and tissue motion. When analysing 2D-images with low resolution moving the stored cine images back and forth usually helps defining the endocardial and epicardial contours. When acquiring functional parameters changes with breathing should be taken in account in the selection for analysis.
21.2.2.3 Anaesthesia
Echocardiography recordings should be as physiological as possible. It is crucial to maintain animal temperature to preserve heart rate and cardiovascular function. With anaesthesia, shaving and application of ECHO gel animals rapidly become hypothermic. If possible the ECHO gel should be heated as well as local environment. In an attempt to reach readings closest to physiology many researchers perform ECHO in restrained unanaesthetised animals usually after previous animal training sessions. The main drawbacks are animal stress, variations in stress response between groups, and poor technical conditions to perform the exam. Other researchers minimally sedate animals and perform the exam under spontaneous ventilation. In these conditions care must be taken to avoid compressing the thorax because the animal’s respiration is already depressed, and the irregular breathing patterns make image acquisition difficult. Another alternative is to perform assisted breathing in order to prevent hypoxia or hypoventilation in a lightly anaesthetised animal without remarkably disturbing cardiovascular physiology. Again the probe should be applied gently to the chest to avoid animal reactions and to minimize cardiovascular reflexes elicited by chest compression such as bradycardia and hypotension. Deep anaesthesia and controlled ventilation are not advisable. As for the anaesthetic to choose, most researchers now prefer halogenated gases due to their easy titration, rapid reversibility and minimal cardiovascular depression.
21.2.2.4 Echocardiographic Views in Rodents
Each researcher will adopt and become familiarized with his/her own protocol but we briefly describe the most standard views. A parasternal long-axis view can easily be obtained (Fig. 21.2A1). In this view it is possible to measure aortic root dimensions and left ventricular (LV) long axis, from the mitral annulus to LV endocardial surface at the apex (Fig. 21.2A1a). Care must be taken not to foreshorten the distance to the apex by ensuring that the longer axis is visualized (Fig. 21.2B1b). The left atrium and mitral valve function can also be gauged in this view and closer to the probe and adjacent to the aorta the right ventricular outflow tract is also visualized. Slightly tilting the probe can reveal the right heart and the pulmonary artery. While both ascending aortic banding and pulmonary artery banding can be easily visualized in this view the alignment for Doppler measurements is only adequate for the pulmonary artery (Fig. 21.2A1b). By rotating the probe 90° a parasternal short axis view is obtained (Fig. 21.2A2), the probe can then be moved gently either cephalad to view first the left and right ventricular outflow tracts (Fig. 21.2A2b), the origin of the great vessels and the aortic and pulmonary valves, then the main pulmonary artery branching (Fig. 21.2A2a) and finally the aortic arch or caudally to view the papillary muscles (Fig. 21.2A2d) and myocardial apex. Fractional shortening, ejection fraction and wall-thickness estimation are usually performed by most researchers with M-mode (Fig. 21.2B2b) with the cursor placed at the longest LV crossing near the tip of the papillary muscles (Fig. 21.2A2c). Slightly tilting the probe usually allows the identification of the roundest LV contour but minimal deviations can result in errors of estimation (Fig. 21.2B1a). Fractional area change can also be computed from 2D-echocardiography at this view with a lower temporal-resolution (Fig. 21.2A2c). Left ventricular mass can be estimated from M-mode tracings or 2D-echocardiography tracings based on distinct geometric assumptions such as the cubed method, the area-length method or the truncated ellipsoid, for a review see Collins et al. [7]. The four chamber view can be obtained by placing the probe where the apex is expected to be and directing the ultrasound beam medially, cephalad and posteriorly to traverse both the LV and right ventricular walls, both atria, and the interatrial and interventricular septa (Fig. 21.23a). This view enables reasonably good orientation of a Doppler mitral and tricuspid flow signal and also the acquisition of tissue Doppler signals near the mitral and tricuspid valve annuli. Is also permits good alignment of the mitral and tricuspid annuli to assess their motion in M-mode (Fig. 21.2B2a). With training the researcher will also realize that a slight deviation or tilting of the probe can usually reveal the left ventricular outflow tract and aorta (Fig. 21.2A3b) in an angle that is convenient for Doppler assessment of velocity of flow (Fig. 21.2B3b). When two orthogonal long-axis views or even combined short and long axis views of the LV are available measurement of areas in systole and diastole allows determination of ejection fraction by a method similar to the biplane, stacked discs or modified Simpson method [8]. Another view that is useful for assessing a successful transverse aortic constriction is the suprasternal view of the aortic arch and its main branches (Fig. 21.2A4a).
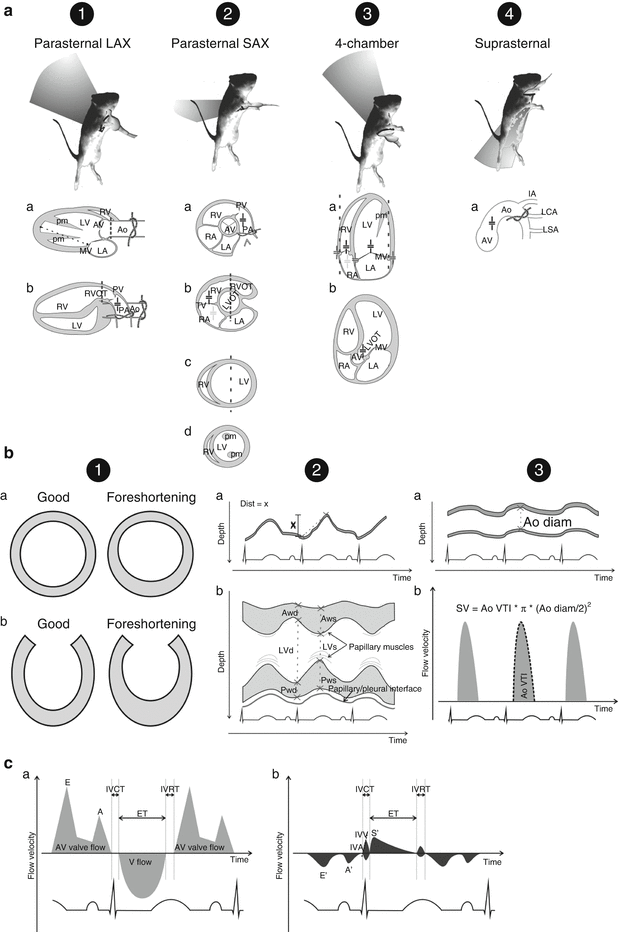
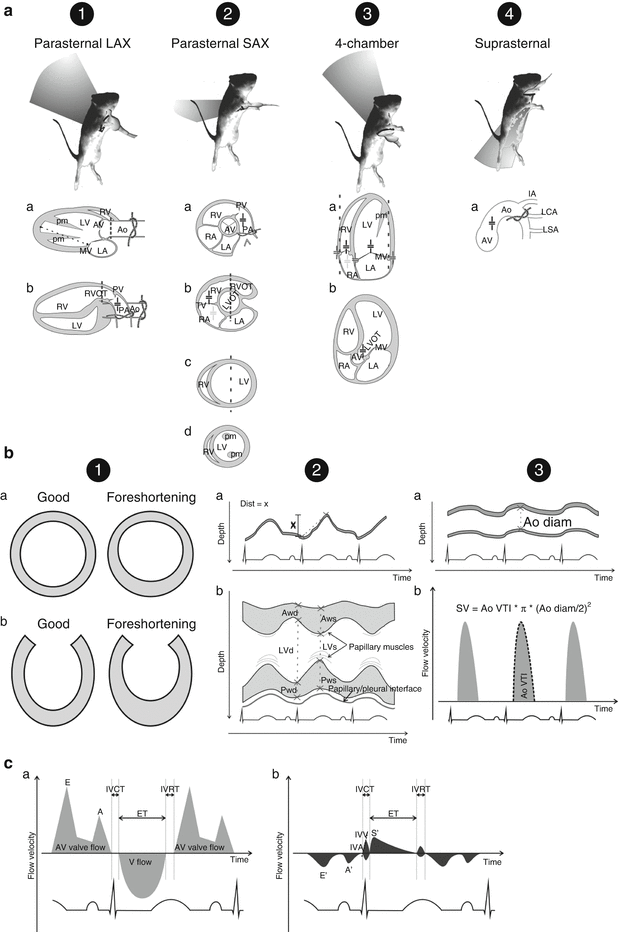
Fig. 21.2
Experimental echocardiography in rodents. Standard echocardiographic views (A): (1) parasternal long-axis (LAX), (2) parasternal short-axis (SAX), (3) 4-chamber view, and (4) suprasternal view Variations in standard views are signalled by a, b, c, and d, see text for details. Typical locations for banding visualization are signalled with a knot; left ventricular long axis dimension measurement is marker in panel 1a; M-mode readings of dimensions or annuli displacement are marked with dashed lines; usual sites for Doppler recordings are indicated by the cursor (+): black, dark grey, and light grey cursors indicate pulsed-wave flow Doppler, pulsed-wave tissue-Doppler Doppler, and continuous-wave Doppler, respectively, dark grey cursor. Ao aorta, AV aortic valve, IA innominate artery, LA left atrium, LCA left common carotid artery, LV left ventricle, LVOT LV outflow tract, LSA left subclavian artery, MV mitral valve, PA pulmonary artery, pm papillary muscle, PV pulmonary valve, RA right atrium, RV right ventricle, RVOT RV outflow tract, TV tricuspid valve. Particular 2D, M–mode and pulsed–wave Doppler recordings (B): (1) illustration on the importance of not foreshortening left ventricular dimensions in short-axis (a) or long-axis (b), (2) M-mode image of atrioventricular valve annulus excursion (dist = x) (a) and left ventricular short axis for fractional-shortening measurement (b), (3) M-mode measurement of aortic dimensions (a) and pulsed-wave Doppler of aortic flow (b). Stroke volume (SV) can be retrieved from the aortic velocity of flow-time integral (Ao VTI) and aortic diameter (Ao diam). AWd and AWs, anterior wall dimension at end-diastole and end-systole, respectively; LVd and LVs, left ventricular cavity dimensions in end-diastole and end-systole, respectively; PWd and PWs, posterior wall dimensions at end-diastole and end-systole, respectively. Atrioventricular (AV) valve flow Doppler (a) and corresponding tissue–Doppler (b) at the myocardium adjoining the annulus (C): ventricular (V) flow usually contaminates the recording enabling the determination of ejection time (ET) in the AV valve flow Doppler. Isovolumetric acceleration (IVA) is estimated as the slope of velocity rise in myocardial motion during isovolumetric contraction. A peak-velocity of late or atrial transAV flow, A′ late velocity of diastolic myocardial motion, E early velocity of transAV flow, E′ early velocity of diastolic myocardial motion, IVCT isovolumetric contraction time, IVRT isovolumetric relaxation time, IVV maximum isovolumetric velocity, S′ maximum velocity of myocardial motion during ejection
21.2.2.5 Functional Indexes
It is beyond the scope of this chapter to describe all research work on functional evaluation by ECHO. We will summarize briefly some of the most commonly employed functional indices. Fractional shortening, fractional area shortening and ejection fraction have already been mentioned. These are highly load dependent indexes and prone to errors in estimation due to their inability to fully scope segmental changes and their reliance on geometrical assumptions of ventricular volumes. Cardiac output can be assessed by multiplying heart rate by stroke volume. Stroke volume can be measured by integrating the velocity of flow over time in an aortic (Fig. 21.2B3b) or LV outflow tract pulsed-wave Doppler acquisition and multiplying it by the cross-sectional area of the aorta (Fig. 21.2B3a) or left ventricular outflow tract, respectively [7]. When there are large differences in animal weight cardiac output should be normalized for body surface area, which is then named cardiac index [9]. The myocardial performance index originally described by Tei et al. evaluates overall LV or right ventricular myocardial function simply based on Doppler-derived intervals without any assumption of volume. It can be calculated from both blood flow Doppler (Fig. 21.2Ca) or tissue Doppler derived readings (Fig. 21.2Cb). Briefly it is derived by dividing the difference of the time from cessation to onset of mitral inflow with duration of ejection by the duration of ejection, or alternatively by dividing the sum of the isovolumetric contraction and relaxation periods (IVCT and IVRT, respectively) by ejection time (ET) [10]:
< div class='tao-gold-member'>
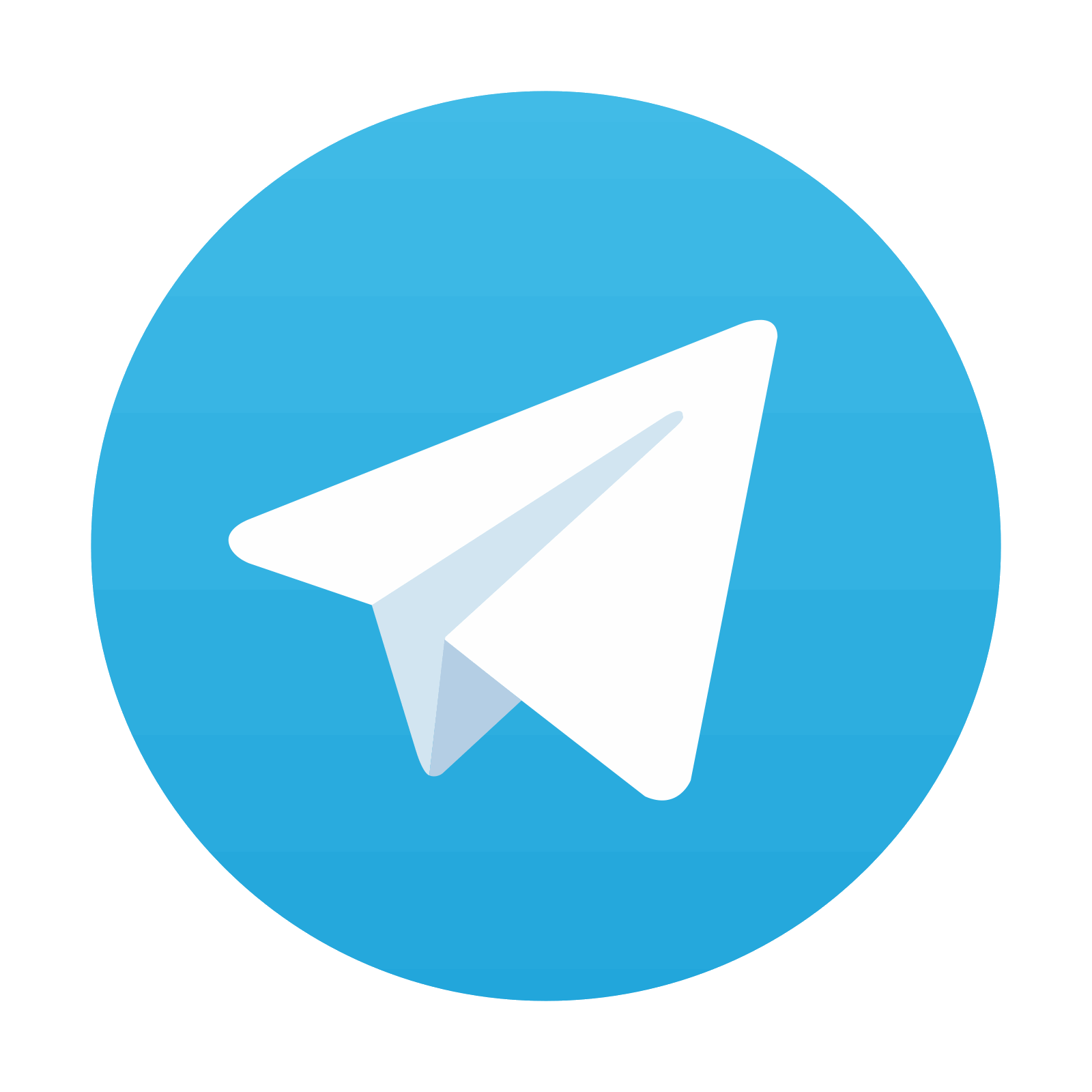
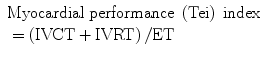
Only gold members can continue reading. Log In or Register a > to continue
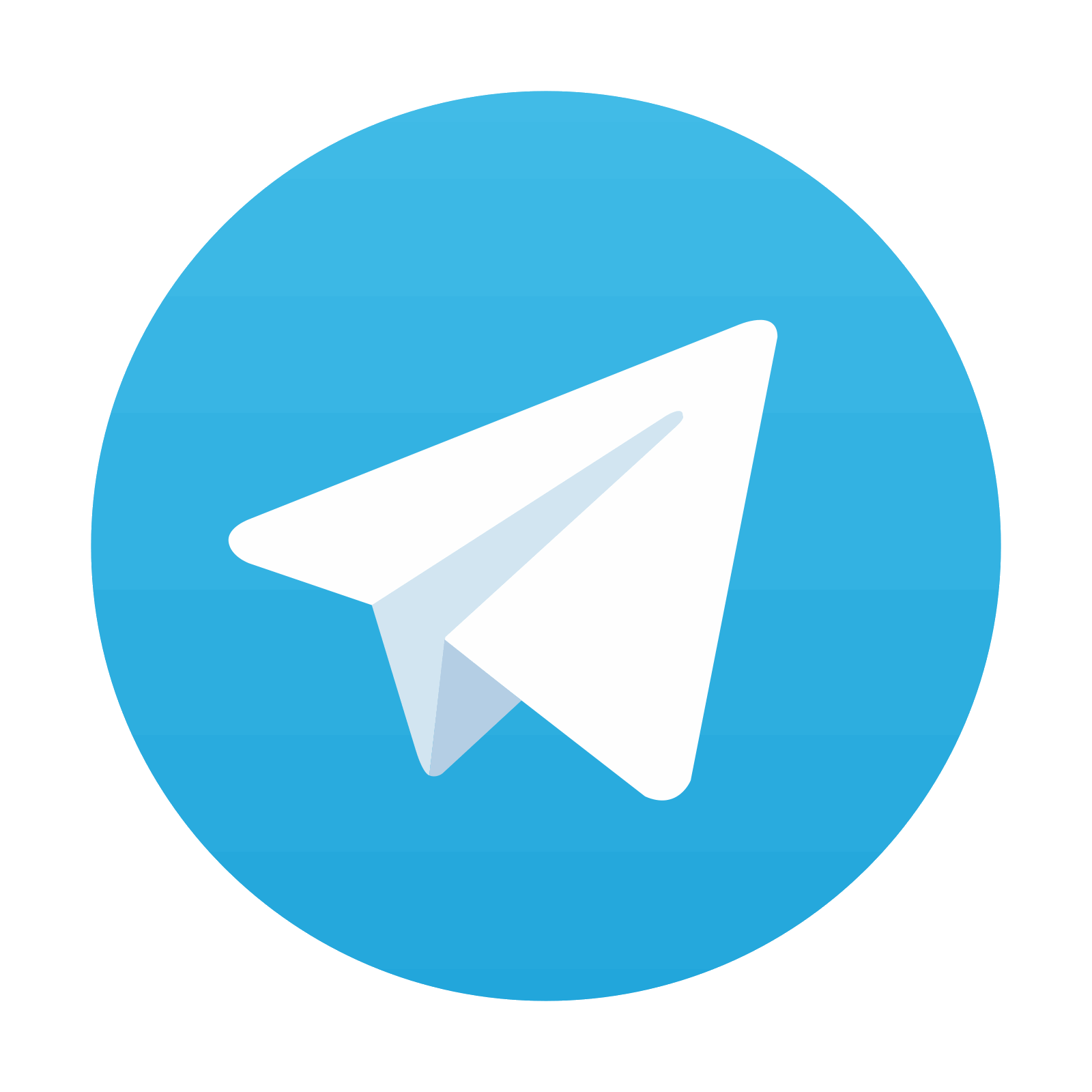
Stay updated, free articles. Join our Telegram channel
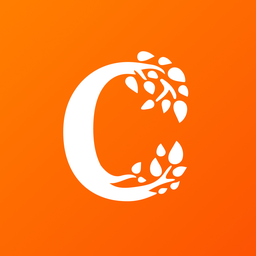
Full access? Get Clinical Tree
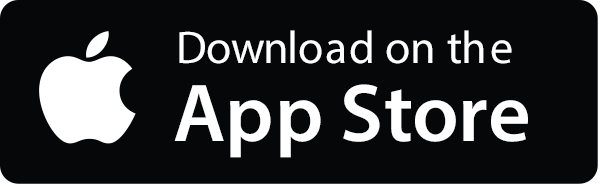
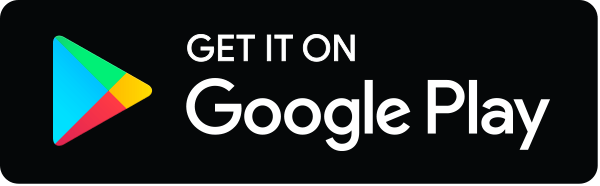
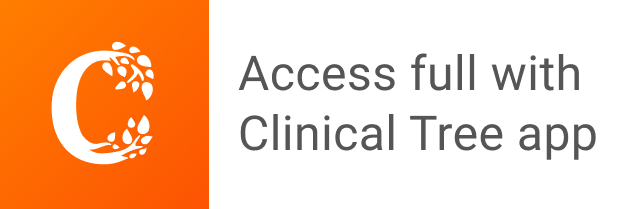