Fig. 2.1
The multi-scale structure of the heart. The function of the heart is dependent on its hierarchical architecture, which spans organ, tissue, cellular, and molecular levels
2.1 Structure–Function Relationships in Single Cardiac Myocytes
The heart consists of millions of individual cardiac myocytes, rhythmically contracting in synchrony to pump blood through the vasculature for the lifetime of the organism. As described in this section, the contractile function of each individual cardiac myocyte is dependent on several intracellular and extracellular factors, including myofibril architecture, myocyte geometry, and the chemical and mechanical properties of the extracellular matrix, each of which can be individually investigated in vitro.
2.1.1 Myofibrillogenesis: Self-Assembly of the Contractile Apparatus
Cardiac myocytes contract because they are densely packed with myofibrils, which consist of repeating sarcomere units that span the long axis of the cell. Each sarcomere consists of interdigitating actin and myosin filaments that slide past each other to shorten the cell and generate contractile forces that sum together to induce organ-level pumping (Boateng and Goldspink 2008). Sarcomeres and myofibrils are highly ordered structures that self-assemble during embryonic and postnatal development by processes that are not well understood, limiting our ability to understand sarcomere dysfunction in disease or replicate mature myofibrillogenesis in engineered cardiac cells and tissues for regenerative medicine.
Much of our knowledge about myofibrillogenesis originates from in situ imaging of developing embryo hearts. Although these studies can reveal how myofibrillar proteins interact and organize in living embryonic hearts (Ehler et al. 1999; Hirschy et al. 2006), tissues inside embryos are difficult to image in real time and thus must be fixed and sectioned, providing only “frozen snapshots” of developmental processes. Thus, in vitro systems are valuable because cells in a dish are more accessible to imaging, allowing us to capture dynamic data about how proteins interact, assemble, and function as myocytes rebuild their cytoskeleton in culture (Sanger et al. 1986; Hilenski et al. 1991; Rhee et al. 1994; Dabiri et al. 1997; Du et al. 2003, 2008). For example, in vitro studies have shown that contractile forces generated by myofibrils are important for maintaining their mature structure (Sharp et al. 1993; Simpson et al. 1993, 1996), indicative of positive feedback between sarcomere maturation and contractility. In silico models have also been developed to test hypotheses about force-dependent myofibril assembly that are impossible or very challenging to test in living systems. A combination of in vitro experiments with computational modeling has shown that myofibril organization is guided by three important phenomena: (1) The geometry of the extracellular matrix available for the cell to bind to its surroundings controls the architecture of its myofibrils, which can serve to organize multiple cells, (2) the amount of force generated by a myofibril increases as it becomes longer, which in turn stabilizes it and promotes its further maturation, and (3) myofibrils couple together laterally to form mutually aligned, parallel bundles of fibers that can maximize contractile forces generated by the cell as a whole (Grosberg et al. 2011b). This type of synergy between in situ, in vitro, and in silico models furthers our understanding of how the highly specialized sarcomeres and myofibrils responsible for myocyte contractility form in the heart.
Understanding myofibrillogenesis is becoming especially important because we now have the technology to differentiate cardiac myocytes from embryonic and induced pluripotent stem cells and progenitor cells (Zhang et al. 2009; Lundy et al. 2013). However, these myocytes often lack the mature striations that are characteristic of primary differentiated cardiac myocytes and, as a consequence, generate significantly lower contractile forces (Domian et al. 2009; Feinberg et al. 2013; Sheehy et al. 2014b), which limits their utility for cell therapy or regenerative medicine. Thus, determining and applying the factors that regulate the structural maturation of myofibrils will be important for directing the maturation of stem cell-derived cardiac myocyte cells and tissues with functional properties comparable to their native counterparts.
2.1.2 Myocyte Shape Remodeling in Development and Disease
Cardiac myocytes in the healthy adult heart are cylindrical, elongated, and densely packed with aligned myofibrils. The rectangular shape of cardiac myocytes emerges over the course of pre- and postnatal development (Hirschy et al. 2006) due to the influence of both intrinsic and extrinsic mechanical forces, such as contractility (Auman et al. 2007) and hemodynamic pressure (Hove et al. 2003). Myocyte shape remodeling is observed in many cardiac diseases, which impacts the morphology of the entire ventricle and its capacity to fill and pump blood. For example, excessive pressure in the ventricle due to factors such as high blood pressure or aortic valve stenosis causes the ventricle to become thicker and hypertrophied (Grossman et al. 1975). This thickening of the ventricle is at least partially attributed to decreases in the length:width aspect ratio of individual cardiac myocytes, which is approximately 7:1 in healthy hearts and decreases to about 4:1 in concentric hypertrophy (Gerdes 2002). In response to volume overload, the ventricle becomes thinner and dilated, known as eccentric hypertrophy (Grossman et al. 1975), and myocyte aspect ratio increases to about 10:1 (Gerdes 2002). Thus, both concentric and eccentric hypertrophy are associated with distinct forms of cellular remodeling that impact gross organ morphology and contribute to contractile dysfunction.
Although we know that distinct changes in myocyte geometry are associated with different cardiac diseases, determining the direct functional implications of cell shape remodeling is challenging because many other parameters are also changing in these diseases. Thus, in vitro systems are useful for isolating the effects of cell shape from other confounding factors. However, cardiac myocytes isolated from the hearts of animals, such as neonatal rats, lose their native architecture in culture and instead adopt arbitrary shapes that are not physiologically relevant (Bray et al. 2008). To control cell shape in culture, extracellular matrix proteins, such as fibronectin, can be micropatterned onto culture substrates with a spatial resolution down to 1 μm using photolithography and microfabrication techniques (Chen et al. 1997). Cardiac myocytes seeded onto these substrates then adhere and spread only on the micropatterned regions of the substrate (Geisse et al. 2009). Using this technique, cardiac myocytes with length:width aspect ratios matching those found in health and disease have been engineered in culture (Fig. 2.2a, b). These studies have revealed that sarcomere organization is regulated by myocyte shape, where myocytes with moderate to high aspect ratios have higher global sarcomere alignment than those with lower aspect ratios (Bray et al. 2008). Thus, optimizing sarcomere alignment is one important consequence of the rectangular shape that cardiac myocytes adopt in the healthy heart.
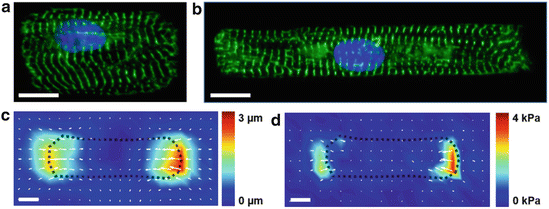
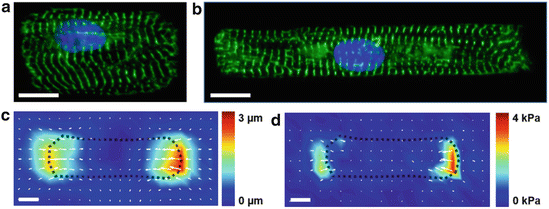
Fig. 2.2
Quantifying structure–function relationships in single cardiac myocytes. The shape of neonatal rat ventricular myocytes can be controlled by seeding cells on micropatterned islands of fibronectin. These myocytes have 2:1 (a) and 7:1 (b) length:width aspect ratios to mimic hypertrophied and healthy myocytes, respectively. Green: alpha-actinin, blue: nuclei. Traction force microscopy can be used to calculate peak systolic displacement (c) and stress (d) in contracting cardiac myocytes cultured on compliant hydrogels. Bars: 10 μm
Another advantage of in vitro systems is that structure–function relationships on the cellular level can be quantified in a controlled setting with techniques such as traction force microscopy (TFM). This method is used to calculate contractile forces generated by cells cultured on compliant hydrogels by tracking the movement of fluorescent beads embedded in the gels (Fig. 2.2c, d) (Butler et al. 2002). TFM studies of micropatterned cardiac myocytes on gels with physiological elasticity have revealed that myocyte shape regulates contractile stress generation in a non-monotonic fashion, with 7:1 aspect ratios generating more peak systolic stress than either lower or higher aspect ratios (Kuo et al. 2012). Thus, the in vivo aspect ratio of myocytes in the healthy heart (7:1) is likely important for maximizing the contractile output of the heart. Calcium cycling was also been found to be regulated by myocyte shape (Kuo et al. 2012), indicating that myocyte shape can impact other functional behaviors such as electrophysiology. Thus, the shape of cardiac myocytes is an important regulator of myocyte phenotype in health and disease.
2.1.3 Extracellular Matrix Regulation of Cardiac Myocyte Structure and Function
Cardiac myocytes are surrounded by an extracellular matrix network with a defined composition that remodels in development (Corda et al. 2000; Williams et al. 2014) and disease (Jane-Lise et al. 2000; Berk et al. 2007). In fetal hearts, the matrix is dominated by fibronectin (Samuel et al. 1994; Farhadian et al. 1995), but over the course of development, fibronectin expression decreases and collagen becomes the primary extracellular matrix protein by adulthood (Borg et al. 1982). Interestingly, many cardiac diseases are associated with reexpression of fibronectin (Farhadian et al. 1995; Ulrich et al. 1997; Konstandin et al. 2013), suggesting that this protein has a role in both developmental and pathological remodeling. Adding to the complexity, signaling from the extracellular matrix is dependent on myocytes expressing the appropriate integrin receptors, which are transmembrane protein complexes that couple specific extracellular matrix proteins to the cytoskeleton (Humphries 2000). Cardiac myocytes express different integrin subtypes throughout development, health, and disease (Terracio et al. 1991; Carver et al. 1994; Nawata et al. 1999; Ross and Borg 2001; Parker and Ingber 2007), which in turn dictates the sensitivity of cardiac myocytes to the evolving composition of the extracellular matrix.
Because both the composition of the extracellular matrix and myocyte integrin expression remodel through cardiac development and disease, these components together are thought to regulate the phenotype of cardiac myocytes. To investigate this, isolated cardiac myocytes have been cultured on substrates coated with different matrix proteins, revealing that matrix composition regulates processes such as signaling (Bullard et al. 2005) and myofibrillogenesis (Hilenski et al. 1991). Proliferation of neonatal rat cardiac myocytes is highest on extracellular matrix isolated from fetal hearts, which is rich in fibronectin, compared to adult matrix, which is primarily collagen (Williams et al. 2014). Adult cardiac myocytes, however, survive only on collagen and laminin, but not fibronectin (Lundgren et al. 1985), consistent with the finding that β-1 integrin, which attaches to fibronectin, is highly expressed in developing (Hornberger et al. 2000) but not adult hearts (Carver et al. 1994). Interestingly, myocytes isolated from rat hearts subjected to volume overload initially show increased adhesion to matrix proteins followed by decreased adhesion (Stewart et al. 2014), indicating that integrin expression and thus interactions with the matrix are dynamic during disease progression. The extracellular matrix can also be used as a cue to differentiate stem cells into cardiac myocytes, as matrices mimicking the native heart have been shown to promote cardiac differentiation of embryonic (Baharvand et al. 2005; Duan et al. 2011) and bone marrow-derived (Sreejit and Verma 2013) stem cells. Thus, the protein composition of the extracellular matrix is an important signal in both cardiac development and disease.
In addition to protein composition, the elasticity of the extracellular matrix also remodels during physiological and pathological growth. In the embryo, the elastic modulus of the heart is approximately 1 kPa and increases to approximately 10 kPa in the adult heart (Engler et al. 2008; Majkut et al. 2013). Many cardiac diseases are associated with fibrosis, which increases the elastic modulus of the tissue to above 50 kPa (Berry et al. 2006; Chaturvedi et al. 2010). To determine how the mechanical properties of the heart regulate myocyte structure and function, investigators have cultured neonatal rat ventricular myocytes on hydrogels with tunable elastic moduli, such as polyacrylamide hydrogels, that have mechanical properties similar to native heart tissue. These studies have shown that sarcomerogenesis and beat rate are optimal at approximately 10 kPa (Engler et al. 2008). At lower elastic moduli, myofibrils do not have enough resistance to generate force and mature (Bajaj et al. 2010). At higher elastic moduli, myocytes contain more stress fibers and less striations (Jacot et al. 2008; Forte et al. 2012). Other functional parameters, such as calcium cycling (Jacot et al. 2008; Galie et al. 2013), are also sensitive to matrix elasticity and are reportedly optimal at an elastic modulus matching the healthy heart. Thus, the mechanical properties of the extracellular matrix play an important role in cardiac myocyte maturation in development and dysfunction in disease.
In concentric hypertrophy, cardiac myocyte aspect ratio decreases (Gerdes 1992) and extracellular matrix elastic modulus increases due to fibrosis (Ho et al. 2010). As described above, both of these factors impact cardiac myocyte structure and function, making it difficult to determine their respective roles in pathogenesis. An advantage of in vitro systems is that we can decouple these two factors by controlling myocyte shape with micropatterning and matrix elasticity with tunable polyacrylamide hydrogels. Using these two techniques, studies have shown that myocytes with healthy length:width aspect ratios (~7:1) generate the most contractile work on gels with healthy elastic moduli (13 kPa), while myocytes with hypertrophied length:width aspect ratios (~2:1) generate the most contractile work on gels with fibrotic elastic moduli (90 kPa) (McCain et al. 2014c). Thus, myocyte shape remodeling in concentric hypertrophy could be the result of myocytes “tuning” their aspect ratio to generate the maximum amount of contractile work for a given matrix elasticity.
2.2 Structure–Function Relationships at the Intercalated Disc
Cardiac tissue functions as an electromechanical syncytium because individual cardiac myocytes are tightly coupled mechanically and electrically by highly specialized cell–cell junctions known as intercalated discs. Intercalated discs consist of adherens junctions, desmosomes, and gap junctions that localize primarily to the short ends of adult cardiac myocytes in ventricular tissue. However, the factors that regulate the assembly, structure, and function of intercalated discs remain mostly elusive, and thus in vitro systems have been useful tools for studying these processes.
2.2.1 Co-development of the Intercalated Disc with the Contractile Apparatus
In situ studies have shown how cell–cell junctions develop relative to other cellular structures, such as the cytoskeleton. In embryonic hearts, myocytes are proliferating and mostly round, myofibrils are immature, and cell–matrix and cell–cell adhesions are uniformly distributed around the cell membrane (Hirschy et al. 2006). In neonatal hearts, myocytes begin to elongate and myofibril striations become more apparent, but both cell–matrix and cell–cell adhesions remain randomly localized around all cell borders (Wu et al. 1999, 2002; Hirschy et al. 2006). By the time the heart reaches full maturity, myofibrils are highly striated and aligned and cell–cell adhesions are localized primarily to the short ends of the myocytes at intercalated disc structures (Angst et al. 1997; Hirschy et al. 2006). Due to the co-development of the contractile apparatus with intercalated discs, one hypothesis is that myofibril development, and thus contractility, might be an important regulator of intercalated disc assembly.
To investigate how mechanical forces regulate cell–cell junction maturation, in vitro systems are used because they allow investigators to probe dynamic structure–function relationships in living cells and tissues. Studies using pairs of noncardiac cells, such as endothelial cells, cultured on compliant hydrogels or flexible microposts have shown that the size of cell–cell junctions is regulated by cytoskeletal tension (Liu et al. 2010; Maruthamuthu et al. 2011). Similar cell pair systems been used to characterize relationships between contractile forces and cell–cell junction formation in beating cardiac myocytes (McCain et al. 2012b). Early in culture, myocyte pairs contain sarcomeres and contract, but much of the contractile force is transmitted to the extracellular matrix adjacent to the cell–cell interface, suggestive of weak mechanical coupling between cells. At this stage of growth, myocytes are also not completely synchronous, indicative of weak electrical cell–cell coupling. Later in culture, the magnitude of contractile force increases and, importantly, force is mostly transmitted across the cell–cell junction instead of to the extracellular matrix. Myocytes also become synchronous, indicating formation of a functional mechanical and electrical syncytium (McCain et al. 2012b). This study shows that, early in tissue morphogenesis, myocytes are primarily adhered to the extracellular matrix, but gradually lose their adhesions to the matrix while increasing adhesion to each other as time progresses. During this process, myofibrils are also actively contracting and maturing, suggesting that adhesion remodeling in the heart is dynamic and occurs even while myocytes are shortening and actively pulling against the cell–cell junction. Studies have also shown that cell–cell junction protein increases in cardiac myocytes exposed to cyclic stretch (Zhuang et al. 2000; Salameh et al. 2010), emphasizing the mechanosensitivity of the intercalated disc. Further studies are needed to better understand the role of mechanical forces in intercalated disc assembly and maturation in developing cardiac tissues.
2.2.2 Potential Role of the Extracellular Matrix in Pathological Intercalated Disc Remodeling
Many cardiac diseases are associated with both cell–cell junction remodeling (Matsushita et al. 1999; Severs 2002; Cabo et al. 2006), which can be arrhythmogenic, and increased fibrosis, which stiffens the myocardium (Doering et al. 1988; Berry et al. 2006; Chaturvedi et al. 2010). Crosstalk between cell–matrix and cell–cell adhesions has been observed in many cell types (Chen et al. 2004; Kim and Asthagiri 2011; Maruthamuthu et al. 2011), suggesting that increased stiffness secondary to fibrosis could induce pathological remodeling of cell–cell adhesions in the heart. To test this, pairs of cardiac myocytes have been cultured on polyacrylamide gels with elastic moduli matching that of a healthy heart and a fibrotic heart (McCain et al. 2012b). On gels mimicking healthy myocardium, myocyte pairs formed mature cell–cell junctions with essentially seamless mechanical communication between cells. Relatively low amounts of force were transmitted to the extracellular matrix. Conversely, on gels mimicking fibrosis, myocytes retained cell–matrix adhesions adjacent to the cell–cell interface and thus a fraction of contractile force was transmitted to the matrix instead of the neighboring cell (McCain et al. 2012b), which potentially disrupts mechanoelectrical coupling between cells. Thus, mechanical properties of the extracellular matrix are potentially propagated to the intercalated disc and could influence cell–cell coupling in healthy and fibrotic hearts.
2.2.3 Engineering Gap Junctions
Gap junction channels facilitate electrical coupling between cardiac myocytes. A single channel consists of two transmembrane connexon hemichannels from neighboring cells, each of which consists of six connexin (Cx) proteins arranged in a hexamer (Noorman et al. 2009). Three Cx isoforms are primarily expressed in the heart: Cx40, Cx43, and Cx45, each of which has its own unique conductance and localization in the heart (Kanter et al. 1992; van Veen et al. 2001). For example, atrial gap junctions consist of Cx40 and Cx43 with small amounts of Cx45. Gap junctions in the ventricle consist mostly of Cx43 with small amounts of Cx45 (Vozzi et al. 1999). Many cardiac diseases are associated with gap junction remodeling, which is thought to underlie arrhythmogenesis by locally changing conductance and propagation velocity (Dupont et al. 2001; Kostin et al. 2003; Yamada et al. 2003; Li et al. 2006; Severs et al. 2006). Thus, determining the factors that regulate the structural and functional properties of gap junctions is important for understanding electrical dysfunction in cardiac disease.
In vitro studies are useful for characterizing the stepwise assembly of protein complexes at the intercalated disc because dissociated cardiac myocytes gradually reform cell–cell junctions in culture, which can be easily imaged (Fig. 2.3a) (Atherton et al. 1986; Hertig et al. 1996; Zuppinger et al. 2000). These studies have shown that adherens junctions and desmosomes, which provide mechanical cell–cell adhesion, form first in developing cardiac tissues. After mechanical adhesion is established, gap junctions form to electrically and chemically couple myocytes together (Kostin et al. 1999; Geisler et al. 2010), indicating a hierarchical relationship where mechanical adhesions precede electrical connections.
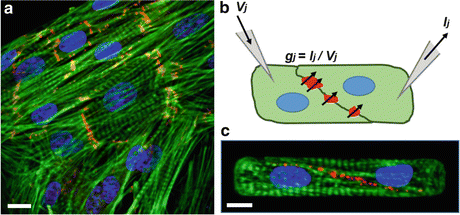
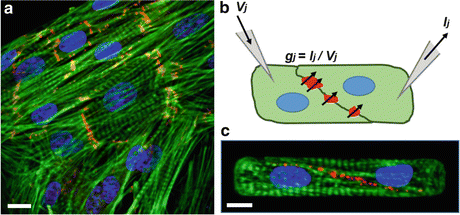
Fig. 2.3
Quantifying structure–function relationships at the intercalated disc. (a) Neonatal rat ventricular myocytes rebuild cell–cell adhesions in culture, providing an easily accessible model system for characterizing intercalated disc assembly. Green: actin, red: β-catenin, blue: nuclei. (b) Dual voltage clamp is used to quantify the electrical conductance of gap junction channels in pairs of cardiac myocytes by applying a voltage (V j ) to one cell, measuring current (I j ) from the second cell, and calculating conductance (g j ). (c) Electrical conductance can then be correlated to gap junction density with immunostaining. Green: actin, red: Cx43, blue: nuclei
Dual voltage clamp is a technique for measuring the electrical conductance between two cultured cells by inserting patch clamp electrodes into each cell, applying a voltage pulse to one cell, measuring current from the second cell, and calculating conductance with Ohm’s law (Fig. 2.3b) (Van Rijen et al. 1998). This technique has traditionally been used on cells randomly plated in culture with little control over the morphology of the cells or the cell–cell junction. By instead culturing myocytes on microcontact printed islands of fibronectin, the size and shape of myocyte pairs can be regulated, as well as cell–cell junction morphology (Pedrotty et al. 2008). For example, neonatal rat ventricular myocytes on micropatterned islands form pairs with highly consistent junction morphologies (Fig. 2.3c), which reduces the variability in functional measurements by controlling for cell–cell contact (McCain et al. 2012a). These studies have shown that electrical conductance between myocytes is affected by the shape of the myocytes (McCain et al. 2012a), which could imply that myocyte shape remodeling in disease could affect gap junction density.
To determine the functional properties of individual gap junction isoforms, investigators have engineered pairs and strands of cardiac myocytes from transgenic Cx knockout mice (McCain et al. 2014b), which have revealed that Cx43 is the primary facilitator of electrical coupling between ventricular myocytes (Beauchamp et al. 2004). The heterogeneous expression of gap junction channels observed in certain pathologies has also been modeled by mixing together Cx43 knockout myocytes with wild-type myocytes in culture (Beauchamp et al. 2012). These studies have reported a significant reduction in Cx43 gap junction formation and electrical coupling between Cx43 knockout and wild-type myocytes on the two-cell level. However, on the tissue level, electrical signals still propagate relatively normally by meandering around the knockout myocytes and finding conduction pathways through wild-type myocytes (Beauchamp et al. 2012). This study highlights the importance of a multi-scale approach because a pathological phenotype, in this case, electrical dysfunction, was apparent on one spatial scale but not another.
Myocytes from Cx knockout mice have also been engineered in culture to determine the function of different gap junction isoforms in the atria. Interestingly, knocking out Cx43 in atrial myocytes decreases propagation velocity, but knocking out Cx40 increases propagation velocity. Knocking out Cx43 also decreases Cx40 expression, but knocking out Cx40 increases Cx43 expression (Beauchamp et al. 2006). Complementary patch clamp studies have shown that Cx43 ablation in atrial myocytes affects the function of sodium ion channels (Desplantez et al. 2012), showing how Cx expression also impacts other protein complexes within the cell. Collectively, these observations indicate that Cx isoforms interact not only with each other but also with ion channels, highlighting the complexity between Cx expression levels and the electrophysiological properties of the tissue.
2.3 Structure–Function Relationships in Multicellular Cardiac Tissues
The electrical and mechanical function of multicellular cardiac tissues is highly dependent on the structure of the tissue. In recent decades, new experimental techniques have been developed to study tissue-level interactions between structure and function in vitro. These experiments have revealed that mimicking the anisotropic structure of healthy heart tissue is essential to fully recapitulating a functioning myocardium, which is a complex and challenging task.
2.3.1 Organization and Structure In Vivo and In Vitro
Structural remodeling of cardiac tissue is evident in many heart diseases, which contributes to functional declines. Two common types of remodeling are seen in the heart: hypertrophy and dilation. Hypertrophy is characterized by thickening of the ventricle walls associated with a disorganization of myocardial tissue. Dilation is characterized by a thinning of the ventricle walls and is also associated with changes in myocyte structure (Ahmad et al. 2005; Ho 2009). Significant regional anatomical remodeling, such as sarcomere disorganization and fibroblast infiltration, can be seen in failing hearts when compared to healthy hearts, further supporting the concept that the structural organization of the heart at multiple scales is important to proper cardiac function (Matsushita et al. 1999; Helm et al. 2006).
To determine how the microscopic organization of cardiac tissue affects its physiological function, several techniques have been developed to control the architecture of cardiac tissues in vitro. One technique is microcontact printing, where myocytes are seeded onto elastic polymer substrates micropatterned with extracellular matrix proteins, such as fibronectin, which induces them to grow along the protein pattern (Tan et al. 2004; Grosberg et al. 2012). A second approach to patterning is to use external topographical cues. Studies have shown that cardiac myocytes organize anisotropically on PDMS scaffolds with repeating ridged features (Kim et al. 2010; Chung et al. 2011) or microgrooved hydrogel substrates (Agarwal et al. 2013a; McCain et al. 2014a). To mimic the multi-scale nature of the heart, other research has produced topographical cues with features that range from micro- to nanoscale by using wrinkled shrink-wrap films as culture substrates (Chen et al. 2011). A final technique to guide organization of cardiac tissue is mechanical stretching. In vitro, cardiac myocytes seeded onto thin PDMS substrates that are cyclically stretched for at least 24 h orient along the direction of stretch (Matsuda et al. 2004; McCain et al. 2013). Thus, several techniques can be used to mimic the architecture of healthy or diseased cardiac tissues in vitro, allowing investigators to study how tissue structure regulates function in a controlled setting.
Although 2D monolayers are helpful for understanding the function of sheets of heart tissue, engineering more complex three-dimensional (3D) tissues is also important for revealing mechanisms of cardiac disease. However, appropriate 3D scaffolds must provide structural and physiological characteristics that mimic that of the heart in vivo. Vascularized cardiac tissue constructs formed by seeding synthetic polylactic acid/poly(lactic-co-glycolic acid) (PLLA/PLGA) porous scaffolds with cardiac myocytes derived from human embryonic stem cells (hESC), endothelial cells, and embryonic fibroblasts have been shown to produce electrophysiological functions similar to those seen in vivo, but do not recreate the anisotropic architecture of native cardiac tissue (Caspi et al. 2007). Rotary jet spun scaffolds of nanofibers have been promising 3D constructs with anisotropic features that promote alignment of cardiac myocytes (Badrossamay et al. 2014), but there is no evidence that these fibers can promote maturation or vascularization. Other studies have shown that electrically pacing neonatal rat cardiac myocytes cultured onto collagen hydrogels produces electrically mature myocytes aligned in the direction of the field lines (Radisic et al. 2004). However, the dimensions of these constructs are currently limited to the depth of the diffusion of oxygen, although adding fluidic channels to the scaffold has been implemented to improve perfusion (Radisic et al. 2008). Thus, although synthetic scaffolds are promising, we still lack the technology to engineer 3D cardiac tissues with proper structural and functional properties.
Recently, decellularized hearts have been used as native cardiac ECM scaffolds, which are perfused and repopulated with cardiac myocytes. Decellularized ECM is advantageous because it maintains all of the necessary structural cues of the native heart. Eight days after reperfusing decellularized hearts with cardiac myocytes, the constructs generate weak pumping function with the aid of electrical stimulation (Ott et al. 2008). Though decellularization followed by repopulation is promising, repopulated hearts are insufficiently mature and revascularization is not possible with current methods. Decellularized mouse hearts have also been repopulated with human iPS-derived cardiac myocytes, demonstrating the potential for decellularized cadaver hearts to serve as scaffolds for a patient’s own cells (Lu et al. 2013). This method would reduce the need for immune suppression after organ transplant. Thus, although engineered 3D cardiac tissues are an attractive “quick fix” to many problems such as heart disease or heart transplant availability, we still do not have the tools to accurately replicate the physiological and mechanical functions of the natural heart.
2.3.2 Tissue Organization and Electrophysiology
In order to efficiently pump blood, the heart relies on a complex network of electrical signals that activates myocytes to contract and relax in a synchronous, pulsatile pattern (Feher 2012). The electrical signals of the heart can be traced in vivo via electrocardiography (ECG) (Guyton and Hall 2000). During infarct, reperfusion, or arrhythmia, the electrical activity of the heart becomes abnormal, which can be detected by ECGs (Zimetbaum and Josephson 2003). Electrophysiological changes have also been observed in diseased hearts with remodeled cardiac tissue. For example, studies have shown that left ventricular hypertrophy, which is characterized by enlarged myocytes, results in lower conduction velocities (Carey et al. 2001). The electrical activity of living hearts can be measured ex vivo using Langendorff preparations, where explanted hearts are immediately perfused with nutrient-rich solutions so that their physiology is preserved outside of the animal’s body. Because Langendorff preparation allows the heart to continue functioning, the electrical properties of the heart can be studied using techniques such as optical mapping (Antz et al. 1998; Efimov et al. 2004; Hucker et al. 2005). Optical mapping entails loading a tissue with a voltage-sensitive dye, which changes its emission properties based on the membrane potential of the tissue. Thus, action potential propagation can be tracked using cameras equipped for high-speed fluorescent imaging. For example, optical mapping of Langendorff preparations has been used to show changes in electrophysiology with respect to molecular changes in the tissue (Morley et al. 1999).
Electrical activity within engineered 2D cardiac monolayers can also be visualized with optical mapping by tracking the fluorescence of voltage-sensitive dyes loaded in tissues that are mounted on a microscope (Fast and Kleber 1993; Bursac et al. 2002). In addition, calcium-sensitive dyes can be used to detect calcium transients within cardiac myocytes and their contributions to atypical beating (Sirenko et al. 2013). Using these methods, it has been shown, in vitro, that electrophysiological abnormalities depend on tissue organization. For example, cardiac tissue alignment has a significant effect on the electrophysiological properties of the heart, such as action potential duration, conduction velocities, and calcium transients (Fast et al. 1996; Bursac et al. 2004; Kim et al. 2010; Chung et al. 2011; Feinberg et al. 2012). Importantly, aligned tissues have a higher longitudinal conduction velocity, which is likely because action potentials propagate more rapidly through cytoplasm than across cell–cell junctions (Spach et al. 2004). These studies indicate that cardiac tissue architecture and electrophysiology are tightly coupled.
2.3.3 Tissue Organization and Contractility
Cardiac contractility is vital to the function of the cardiovascular system. The contraction of the heart can be analyzed at various scales ranging from the cellular scale to the full organ scale (Humphrey 2010). Changes in heart contractility are usually associated with poor pumping function, which are signs of a diseased heart (Guyton and Hall 2000). Ejection fraction is a measure of the volumetric fraction of blood leaving the heart with each contraction and is typically 55 % or greater during systole in a normal healthy heart (Wackers et al. 1979). The contraction during systole is characterized by a 14 % shortening of the myofibers accompanied by a significant thickening of the heart wall (Chen et al. 2005). Additionally, pressure changes within the chambers themselves act as secondary driving forces to transport blood into and out of the chambers, which aids contractility.
Heart disease is highly correlated with changes in contractility, as measured by changes in ejection fraction. For example, hypertrophy, characterized by an enlarged heart, is associated with a decrease in ejection fraction (Feild et al. 1973). Using the Langendorff preparations previously mentioned, it has been shown that ischemic hearts have much higher diastolic pressures than healthy, non-ischemic hearts (Mirica et al. 2009). To begin to understand what factors are contributing to poor function of the diseased heart, studies that elucidate these elements on a smaller scale must first be conducted.
< div class='tao-gold-member'>
Only gold members can continue reading. Log In or Register a > to continue
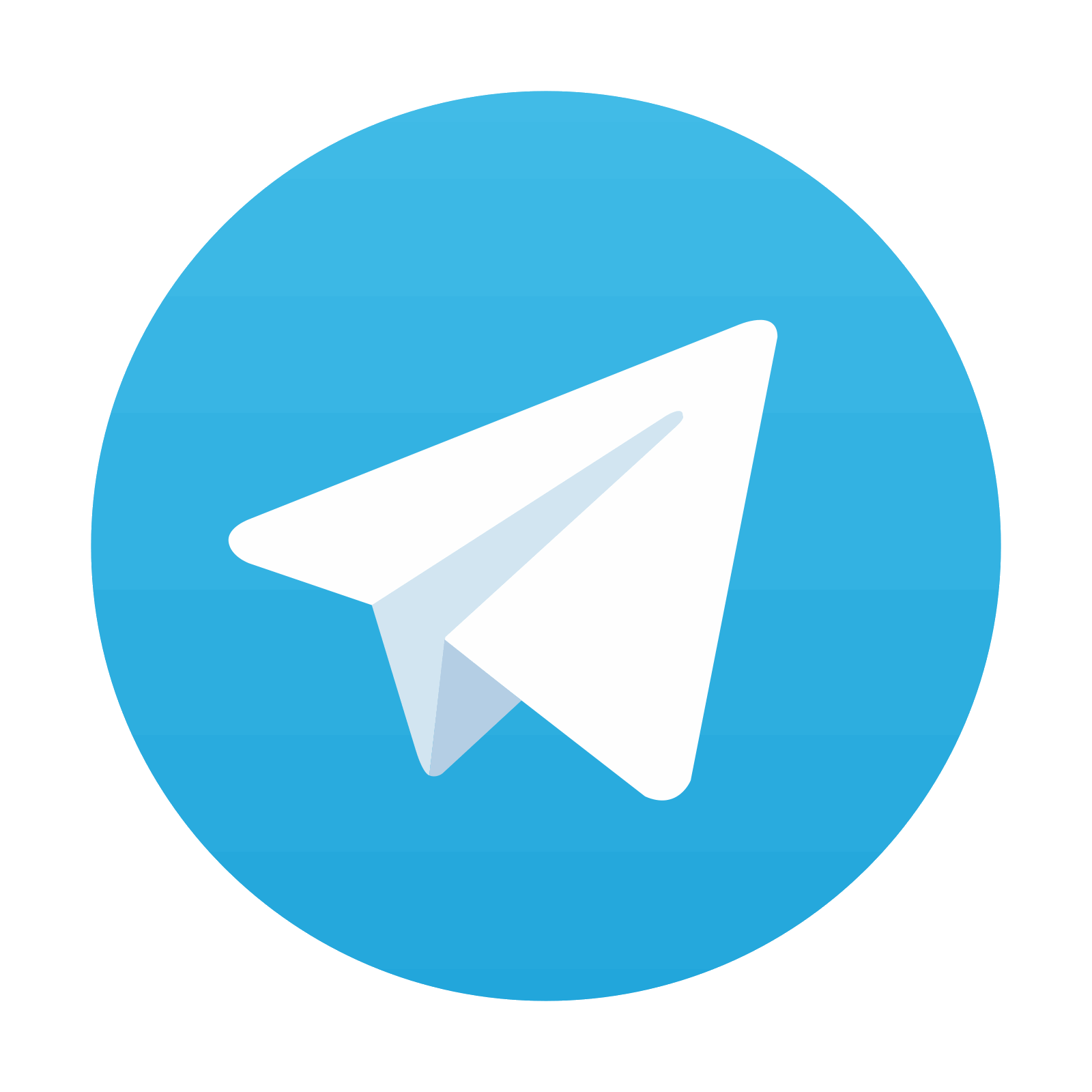
Stay updated, free articles. Join our Telegram channel
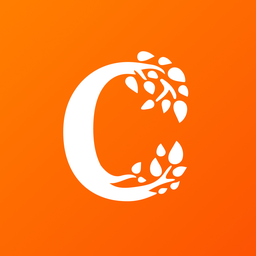
Full access? Get Clinical Tree
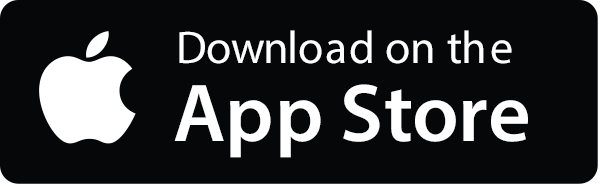
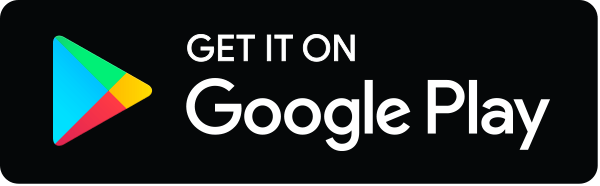