Abstract
Background/Purpose
Biodegradable polymers are the main materials for coronary scaffolds. Magnesium has been investigated as a potential alternative and was successfully tested in human clinical trials. However, it is still challenging to achieve mechanical parameters comparative to permanent bare metal (BMS) and drug-eluting stents (DES). As such, in vitro tests are required to assess mechanical parameters correlated to the safety and efficacy of the device.
Methods/Materials
In vitro bench tests evaluate scaffold profiles, length, deliverability, expansion behavior including acute elastic and time-dependent recoil, bending stiffness and radial strength. The Absorb GT1 (Abbott Vascular, Temecula, CA), DESolve (Elixir Medical Corporation, Sunnyvale, CA) and the Magmaris (BIOTRONIK AG, Bülach, Switzerland) that was previously tested in the BIOSOLVE II study, were tested.
Results
Crimped profiles were 1.38 ± 0.01 mm (Absorb GT1), 1.39 ± 0.01 mm (DESolve) and 1.44 ± 0.00 mm (Magmaris) enabling 6F compatibility. Trackability was measured depending on stiffness and force transmission (pushability). Acute elastic recoil was measured at free expansion and within a mock vessel, respectively, yielding results of 5.86 ± 0.76 and 5.22 ± 0.38% (Absorb), 7.85 ± 3.45 and 9.42 ± 0.21% (DESolve) and 5.57 ± 0.72 and 4.94 ± 0.31% (Magmaris). Time-dependent recoil (after 1 h) was observed for the Absorb and DESolve scaffolds but not for the Magmaris. The self-correcting wall apposition behavior of the DESolve did not prevent time-dependent recoil under vessel loading.
Conclusions
The results of the suggested test methods allow assessment of technical feasibility based on objective mechanical data and highlight the main differences between polymeric and metallic bioresorbable scaffolds.
1
Introduction
Transcatheter angioplasty and stenting has developed at an incredible pace starting with the famous first attempts of Dotter, Grüntzig, Sigwart, Puel and others . Several generations of balloon catheters and stents have evolved. Drug-eluting stents (DES), either with biodegradable polymers or polymer-free coating, are the current standard of care in the field of cardiovascular intervention. They were successfully developed with the intention to help reduce the incidence of neointimal hyperplasia and in-stent restenosis . The introduction of completely bioresorbable scaffolds hopes to overcome remaining limitations of permanent implants such as the risk of late stent thrombosis or strut fracture-induced restenosis, impaired lesion imaging with computed tomography or magnetic resonance, complication of repeated treatments to the same site and restricted vasomotion or side-branch access long-term .
Indeed, the idea of bioresorbable scaffolds was recently made real by marketing of two polymer based scaffolds (Absorb GT1 and DESolve). One metallic, magnesium based bioabsorbable scaffold was also successfully tested and approved for clinical studies. Several reviews are available describing the current state of technology. In most of these cases, the word “stent” was replaced by “scaffold” to emphasize the temporary character of the new product class. The process of bioabsorption has been described with the words biodegradation or biocorrosion depending on the polymer or metal material used. Bioabsorption or bioresorption will be used as synonyms.
Despite the advanced state of the bioresorbable scaffold technology, properties influencing safety and efficacy have yet to be published.
Main properties include: crimped scaffold diameter and strut thickness but also more complex functional parameters such as deployment behavior, length change during deployment, elastic recoil, radial strength and bending stiffness of the expanded scaffolds. Deliverability, expressed as a function of trackability and pushability, is also an important parameter influencing the safety and efficacy of the device. Moreover, since most of the scaffolds are polymeric, effects of viscoelasticity or time-dependent diameter changes have to be considered. Beyond these characteristics, more detailed investigations may be needed to assess particular properties resulting from the use of bioabsorbable materials.
It is the goal of this paper to describe and discuss relevant test methods, conducted both on polymeric and metallic bioresorbable scaffolds, and to discuss their respective results.
2
Methods
2.1
Geometrical data
2.1.1
Crimped profile
The crimped profile describes the diameter of the unexpanded scaffold on the dilatation balloon. It is determined using a non-contact method consisting of a 2-axis laser scanner (ODAC 64 XY, Zumbach Electronic AG, Orpund, Switzerland) as part of a specific setup for stent testing . The laser scanner measures the diameter of the catheter and mounted stent in two perpendicular directions (x, y). Assuming cylindrical cross sections the profile at each measurement point (step width 0.2 mm along the distal part of the stent systems) is summarized as root–mean–square (RMS) value. For comparison the mean profile of the crimped scaffold is derived by averaging. The non-contact method avoids any potential influence of the method itself during the measurement and insures that the 2% accuracy required (or even 1% ) can be achieved. The accuracy is critical for small diameters as for the ones encountered with coronary stent systems. Indeed, for a stent profile of 1.000 mm the absolute diameter error has to be in the range of 0.020 mm (0.010 mm) including all uncertainties of measurement and calibration.
2.1.2
Scaffold length
Changes in stent length are observed during implantation procedure, in particular during stent expansion. Compared to the crimped profile measure, length measurement does not have the same challenging requirements. However, contactless measurement is still preferred. The setup for diameter measurements as mentioned above can be used for determination of scaffold length before and after scaffold expansion together with the measurement of the diametric dimensions. After positioning a cross line at both ends of the scaffold (crimped or expanded) the length is automatically calculated and saved.
2.1.3
Strut dimensions
Strut dimensions directly contribute to the safety and efficacy of the device as well as to its mechanical properties . Despite the tendency to lower strut thickness for permanent stents, limitations arising from the materials chosen for bioabsorbable scaffolds have to be accepted . Strut thickness is reported by manufacturers and is expected to be stable. The measurement requires high-resolution imaging such as scanning electron microscopy combined with a calibrated scale.
2.2
Scaffold system delivery
The deliverability of stent or scaffold systems, characterized by functional parameters like trackability and pushability, is one of the clinically most important requirements . The visibility of the scaffold during intervention as well as the healing period is equally important.
2.2.1
Trackability
The trackability describes the ability of a system to be advanced through a curved vessel anatomy. The analyses of trackability were done using a special measurement arrangement, the 2-channel PUSH device. The system is able to measure proximal and distal forces during the passage of the stent system through the guiding catheter and the vessel model. For measurements the test arrangement was combined with a guiding catheter Vista brite tip 6F JL 4 (Cordis Corp., Freemont, CA, USA) and a guide wire Galeo M 0.014″ (BIOTRONIK AG, Bülach, Switzerland). The guide wire was changed after tracking 10 systems. The guiding catheter was used for all test samples. The investigations were carried out in a vessel model placed inside a heated water bath of 37 ± 2 °C and the stent system was inserted at a proximal traverse speed of 7.5 mm/s ( Fig. 1 ). The relevance of this model was shown in previous studies . For trackability the proximal push force was measured using a load cell (type 3482, burster GmbH&Co. KG, Gernsbach, Germany, measurement range ± 5 N). Each stent system was measured 3 times for each test. The resulting force–distance curves were recorded and analyzed for maximum and average track forces.
2.2.2
Pushability
The pushability is a functional parameter to assess the force transfer from the proximal shaft to the very distal tip of the stent system. Maximum transfer is limited by catheter flexibility. High pushability provides optimal feedback to the user and thus enables safe catheter maneuvers. Additionally, optimum pushability has a positive impact on catheters trackability . Pushability was measured using the trackability setup , however, a simulated vessel curvature was added by a total occlusion model at the end. The occlusion model was fixed at a load cell (type 3482, burster GmbH&Co. KG, Gernsbach, Germany, measurement range ± 2 N) measuring the distal reaction force F dist . The arrangement was placed in 37 °C heated water. The delivery system to be tested was fixed with its proximal hub at a linear motor drive and a load cell measuring the proximal push force F prox during automatic advancement of the system. Both forces were recorded and the ratio of F dist /F prox was calculated at F prox ≈ 3.5 N as the measure of pushability.
2.3
Radiopacity
Visibility of vascular implants is an important requirement for a safe and effective application. Despite developing non-ionizing imaging modalities such as magnetic resonance imaging (MRI), intravascular ultrasound (IVUS) or optical coherence tomography (OCT), visibility of implants still depends on their radiopacity (X-ray attenuation) . The measurement of radiopacity as defined by ASTM F640–12 is “determined by ( a ) qualitatively comparing image(s) of a test specimen and a user-defined standard, …, or ( b ) quantitatively determining the specific difference in optical density or pixel intensity between the image of a test specimen and the image of a user-defined standard, …” .
The radiopacity of balloon markers, scaffold markers (commonly available on bioabsorbable scaffolds) and of the scaffolds themselves was measured using an X-ray device (Bucky Diagnost, Philips, Amsterdam, The Netherlands) based on a storage film system (MC10, Agfa HealthCare GmbH, Cologne, Germany) with standardized imaging parameters (70 kV). The basic setup was used according to (tube – film distance 115 cm, 150 mm PMMA phantom). Additionally to the test objects an aluminum (Al) sample (thickness 1 to 10 mm, in steps of 1 mm) was placed on the scattering phantom as a reference.
According to Ref. the radiopacity is calculated as the difference between the gray scale values of the investigated region and the adjacent image area. Comparison with the Al sample provides similar results.
2.4
Mechanics of scaffolds
2.4.1
Scaffold expansion
In vitro investigation of scaffold expansion is necessary to describe the behavior of pressurized balloon and scaffold using measurement options far beyond clinical imaging modalities in a well-controlled laboratory environment . In general, heated water or saline at 37 ± 2 °C is used to simulate body temperature. This is relevant for all balloon materials affecting balloon strength and compliance, but also for polymer based scaffolds. In particular the material strength and elongation at break strongly depend on temperature and humidity.
In addition to the features for profile measurement, a computer driven pressure regime has to be realized for the balloon. For characteristics of the used equipment see Table 1 .
Laser | 2 axis measurement head ODAC 64XY-RSN (ZUMBACH) |
Measurement range | 0.1 … 30 mm |
Measurement accuracy | ± 0.01 mm |
Resolution | ± 0.001 mm |
Temperature | 37 ± 2 °C |
Pressure control unit | neMESYS (cetoni GmbH, Korbußen, Germany) |
Pressure range | 1 … 40 bar (1 atm = 101.325 kPa = 1.01325 bar) |
Accuracy | < 5% of measured value |
Resolution | 0.05 bar |
Compliance of the scaffold system (pressure-diameter characteristic) and elastic recoil can be derived from profile data during scaffold expansion and subsequent balloon deflation.
2.4.2
Elastic recoil
The elastic recoil is of special interest for bioabsorbable scaffolds. Considering viscoelasticity under constant loading and creeping/relaxation of polymers after discontinuation of loading, time dependent effects of diameter change have to be investigated . Our study considered two methods for measurement of recoil ( Fig. 2 ):
(a) Recoil without outer loading ( Fig. 2 a).
The scaffold expansion was done manually with the help of an inflation/deflation device (20/30 PRIORITY PACK Accessory Kit, Abbott) into a rigid vessel model made from PMMA (half shell model with a vessel diameter of 3.0 mm). The vessel model was stored in a basin with heated water (37 ± 2 °C). The expansion procedure and time regime was adapted according to the individual instructions for use (IFU).
BIOTRONIK Magmaris
- –
preconditioning the scaffold in simulated body fluid (SBF, 37 ± 2 °C) for 30 s
- –
scaffold expansion in one step to the nominal pressure (10 atm)
Abbott Absorb GT1
- –
Storing the distal part of the catheter in the heated water basin for 60 s
- –
Scaffold expansion in 2 atm increments every 5 s to the nominal pressure (7 atm)
Elixir DESolve
- –
Storing the distal part of the catheter in the heated water basin for 60 s
- –
Scaffold expansion up to 2 atm with 10 s intervals per atmosphere
- –
Continued inflation up to the nominal pressure (9 atm) in 2 s intervals per atmosphere
The target pressure was held for 30 s in all cases, before the balloon catheter was evacuated and removed.
Directly after removing the balloon catheter the scaffold was extracted from the half shell vessel model and set into the constant temperature water bath of the laser test system. The scaffold profile was determined immediately after expansion (acute recoil) and after 1 h (time-dependent recoil).
(b) Recoil with outer loading through mock vessel ( Fig. 2 b).
Three scaffolds per type were expanded into a mock vessel (inner diameter 2.7 ± 0.2 mm, radial compliance 5–7% per 100 mmHg @ 72 bpm, Dynatec Labs, Inc.,Galena, MO, USA). The mock vessel (40 mm long) was placed inside the water bath of the laser test system. The scaffold expansion procedure into the mock vessel was according IFU as described before. To determine the recoil and the scaffold length change, the profile of the mock vessel in the region of the implanted scaffold was measured with the pressurized balloon inside (pressure = NP), after 30 s dwell time and removal of the balloon (for determination of acute recoil) and after one hour (for determination of time-dependent recoil). Between the measurements the position of the mock vessel and the scaffold was not changed.
2.4.3
Radial strength
The main and initial function of expanded stents or scaffolds is to support the dilated vessel and prevent from partial prolapse of tissue. In this context, standardization and guidance literature requires testing of radial strength, which is a measure for crush resistance of expanded stents. It was determined using the 2-axis laser test system as described for the crimped profile measurement. The scaffold was expanded into a thin polyurethane tube (PUR, wall thickness: 0.75 mm) fixed to a sample holder and placed into the sealed test chamber. Pressurization of the test chamber was accomplished using the computer-driven pressure control unit. For the presented investigations, scaffold expansion directly into the test tube was manually done with the help of an inflation device following the regime according to manufacturer’s IFU. The test chamber was completely filled with temperature controlled water (37 °C) and connected with a tubing system to the pressure control unit. The pressure in the test chamber was incrementally increased by steps of 2 kPa to 300 kPa. This applied a radial force on the PUR-tube around the scaffold. The pressure at which the scaffold lost its support function was noted as the collapse pressure.
2.4.4
Flexibility/Bending stiffness
The bending stiffness is a measure of a structure’s resistance to bending deformation. It is the reciprocal of flexibility . The bending stiffness of the balloon section with mounted scaffold was measured using an experimental setup where the test sample is fixed at the free bending length l and deflected by the distance f . The resulting bending force F (load cell PW4MC3/300G-1, Hottinger Baldwin Messtechnik GmbH, Darmstadt, Germany, measurement range ± 3 N) is measured for small deflections. The force–distance curves describe the spring modulus of the test objects for bending. The bending stiffness EI is calculated taking the mean value of the slope F/f calculated by linear regression from the whole force–distance curve (beam theory). Considering possible asymmetric structures of the test samples, the bending stiffness was measured in five directions around the circumference and subsequently averaged. The bending stiffness was also measured for expanded scaffolds where a low stiffness is assumed to enable easy adaptation to vessel curvatures.
2.4.5
Statistics
Pooled variance t-test for independent samples (IBM SPSS Statistics 22, IBM Corp., Armonk, NY, USA) was used for statistical analysis. The significance level was set as p < 0.05.
2
Methods
2.1
Geometrical data
2.1.1
Crimped profile
The crimped profile describes the diameter of the unexpanded scaffold on the dilatation balloon. It is determined using a non-contact method consisting of a 2-axis laser scanner (ODAC 64 XY, Zumbach Electronic AG, Orpund, Switzerland) as part of a specific setup for stent testing . The laser scanner measures the diameter of the catheter and mounted stent in two perpendicular directions (x, y). Assuming cylindrical cross sections the profile at each measurement point (step width 0.2 mm along the distal part of the stent systems) is summarized as root–mean–square (RMS) value. For comparison the mean profile of the crimped scaffold is derived by averaging. The non-contact method avoids any potential influence of the method itself during the measurement and insures that the 2% accuracy required (or even 1% ) can be achieved. The accuracy is critical for small diameters as for the ones encountered with coronary stent systems. Indeed, for a stent profile of 1.000 mm the absolute diameter error has to be in the range of 0.020 mm (0.010 mm) including all uncertainties of measurement and calibration.
2.1.2
Scaffold length
Changes in stent length are observed during implantation procedure, in particular during stent expansion. Compared to the crimped profile measure, length measurement does not have the same challenging requirements. However, contactless measurement is still preferred. The setup for diameter measurements as mentioned above can be used for determination of scaffold length before and after scaffold expansion together with the measurement of the diametric dimensions. After positioning a cross line at both ends of the scaffold (crimped or expanded) the length is automatically calculated and saved.
2.1.3
Strut dimensions
Strut dimensions directly contribute to the safety and efficacy of the device as well as to its mechanical properties . Despite the tendency to lower strut thickness for permanent stents, limitations arising from the materials chosen for bioabsorbable scaffolds have to be accepted . Strut thickness is reported by manufacturers and is expected to be stable. The measurement requires high-resolution imaging such as scanning electron microscopy combined with a calibrated scale.
2.2
Scaffold system delivery
The deliverability of stent or scaffold systems, characterized by functional parameters like trackability and pushability, is one of the clinically most important requirements . The visibility of the scaffold during intervention as well as the healing period is equally important.
2.2.1
Trackability
The trackability describes the ability of a system to be advanced through a curved vessel anatomy. The analyses of trackability were done using a special measurement arrangement, the 2-channel PUSH device. The system is able to measure proximal and distal forces during the passage of the stent system through the guiding catheter and the vessel model. For measurements the test arrangement was combined with a guiding catheter Vista brite tip 6F JL 4 (Cordis Corp., Freemont, CA, USA) and a guide wire Galeo M 0.014″ (BIOTRONIK AG, Bülach, Switzerland). The guide wire was changed after tracking 10 systems. The guiding catheter was used for all test samples. The investigations were carried out in a vessel model placed inside a heated water bath of 37 ± 2 °C and the stent system was inserted at a proximal traverse speed of 7.5 mm/s ( Fig. 1 ). The relevance of this model was shown in previous studies . For trackability the proximal push force was measured using a load cell (type 3482, burster GmbH&Co. KG, Gernsbach, Germany, measurement range ± 5 N). Each stent system was measured 3 times for each test. The resulting force–distance curves were recorded and analyzed for maximum and average track forces.
2.2.2
Pushability
The pushability is a functional parameter to assess the force transfer from the proximal shaft to the very distal tip of the stent system. Maximum transfer is limited by catheter flexibility. High pushability provides optimal feedback to the user and thus enables safe catheter maneuvers. Additionally, optimum pushability has a positive impact on catheters trackability . Pushability was measured using the trackability setup , however, a simulated vessel curvature was added by a total occlusion model at the end. The occlusion model was fixed at a load cell (type 3482, burster GmbH&Co. KG, Gernsbach, Germany, measurement range ± 2 N) measuring the distal reaction force F dist . The arrangement was placed in 37 °C heated water. The delivery system to be tested was fixed with its proximal hub at a linear motor drive and a load cell measuring the proximal push force F prox during automatic advancement of the system. Both forces were recorded and the ratio of F dist /F prox was calculated at F prox ≈ 3.5 N as the measure of pushability.
2.3
Radiopacity
Visibility of vascular implants is an important requirement for a safe and effective application. Despite developing non-ionizing imaging modalities such as magnetic resonance imaging (MRI), intravascular ultrasound (IVUS) or optical coherence tomography (OCT), visibility of implants still depends on their radiopacity (X-ray attenuation) . The measurement of radiopacity as defined by ASTM F640–12 is “determined by ( a ) qualitatively comparing image(s) of a test specimen and a user-defined standard, …, or ( b ) quantitatively determining the specific difference in optical density or pixel intensity between the image of a test specimen and the image of a user-defined standard, …” .
The radiopacity of balloon markers, scaffold markers (commonly available on bioabsorbable scaffolds) and of the scaffolds themselves was measured using an X-ray device (Bucky Diagnost, Philips, Amsterdam, The Netherlands) based on a storage film system (MC10, Agfa HealthCare GmbH, Cologne, Germany) with standardized imaging parameters (70 kV). The basic setup was used according to (tube – film distance 115 cm, 150 mm PMMA phantom). Additionally to the test objects an aluminum (Al) sample (thickness 1 to 10 mm, in steps of 1 mm) was placed on the scattering phantom as a reference.
According to Ref. the radiopacity is calculated as the difference between the gray scale values of the investigated region and the adjacent image area. Comparison with the Al sample provides similar results.
2.4
Mechanics of scaffolds
2.4.1
Scaffold expansion
In vitro investigation of scaffold expansion is necessary to describe the behavior of pressurized balloon and scaffold using measurement options far beyond clinical imaging modalities in a well-controlled laboratory environment . In general, heated water or saline at 37 ± 2 °C is used to simulate body temperature. This is relevant for all balloon materials affecting balloon strength and compliance, but also for polymer based scaffolds. In particular the material strength and elongation at break strongly depend on temperature and humidity.
In addition to the features for profile measurement, a computer driven pressure regime has to be realized for the balloon. For characteristics of the used equipment see Table 1 .
Laser | 2 axis measurement head ODAC 64XY-RSN (ZUMBACH) |
Measurement range | 0.1 … 30 mm |
Measurement accuracy | ± 0.01 mm |
Resolution | ± 0.001 mm |
Temperature | 37 ± 2 °C |
Pressure control unit | neMESYS (cetoni GmbH, Korbußen, Germany) |
Pressure range | 1 … 40 bar (1 atm = 101.325 kPa = 1.01325 bar) |
Accuracy | < 5% of measured value |
Resolution | 0.05 bar |
Compliance of the scaffold system (pressure-diameter characteristic) and elastic recoil can be derived from profile data during scaffold expansion and subsequent balloon deflation.
2.4.2
Elastic recoil
The elastic recoil is of special interest for bioabsorbable scaffolds. Considering viscoelasticity under constant loading and creeping/relaxation of polymers after discontinuation of loading, time dependent effects of diameter change have to be investigated . Our study considered two methods for measurement of recoil ( Fig. 2 ):
(a) Recoil without outer loading ( Fig. 2 a).
The scaffold expansion was done manually with the help of an inflation/deflation device (20/30 PRIORITY PACK Accessory Kit, Abbott) into a rigid vessel model made from PMMA (half shell model with a vessel diameter of 3.0 mm). The vessel model was stored in a basin with heated water (37 ± 2 °C). The expansion procedure and time regime was adapted according to the individual instructions for use (IFU).
BIOTRONIK Magmaris
- –
preconditioning the scaffold in simulated body fluid (SBF, 37 ± 2 °C) for 30 s
- –
scaffold expansion in one step to the nominal pressure (10 atm)
Abbott Absorb GT1
- –
Storing the distal part of the catheter in the heated water basin for 60 s
- –
Scaffold expansion in 2 atm increments every 5 s to the nominal pressure (7 atm)
Elixir DESolve
- –
Storing the distal part of the catheter in the heated water basin for 60 s
- –
Scaffold expansion up to 2 atm with 10 s intervals per atmosphere
- –
Continued inflation up to the nominal pressure (9 atm) in 2 s intervals per atmosphere
The target pressure was held for 30 s in all cases, before the balloon catheter was evacuated and removed.
Directly after removing the balloon catheter the scaffold was extracted from the half shell vessel model and set into the constant temperature water bath of the laser test system. The scaffold profile was determined immediately after expansion (acute recoil) and after 1 h (time-dependent recoil).
(b) Recoil with outer loading through mock vessel ( Fig. 2 b).
Three scaffolds per type were expanded into a mock vessel (inner diameter 2.7 ± 0.2 mm, radial compliance 5–7% per 100 mmHg @ 72 bpm, Dynatec Labs, Inc.,Galena, MO, USA). The mock vessel (40 mm long) was placed inside the water bath of the laser test system. The scaffold expansion procedure into the mock vessel was according IFU as described before. To determine the recoil and the scaffold length change, the profile of the mock vessel in the region of the implanted scaffold was measured with the pressurized balloon inside (pressure = NP), after 30 s dwell time and removal of the balloon (for determination of acute recoil) and after one hour (for determination of time-dependent recoil). Between the measurements the position of the mock vessel and the scaffold was not changed.
2.4.3
Radial strength
The main and initial function of expanded stents or scaffolds is to support the dilated vessel and prevent from partial prolapse of tissue. In this context, standardization and guidance literature requires testing of radial strength, which is a measure for crush resistance of expanded stents. It was determined using the 2-axis laser test system as described for the crimped profile measurement. The scaffold was expanded into a thin polyurethane tube (PUR, wall thickness: 0.75 mm) fixed to a sample holder and placed into the sealed test chamber. Pressurization of the test chamber was accomplished using the computer-driven pressure control unit. For the presented investigations, scaffold expansion directly into the test tube was manually done with the help of an inflation device following the regime according to manufacturer’s IFU. The test chamber was completely filled with temperature controlled water (37 °C) and connected with a tubing system to the pressure control unit. The pressure in the test chamber was incrementally increased by steps of 2 kPa to 300 kPa. This applied a radial force on the PUR-tube around the scaffold. The pressure at which the scaffold lost its support function was noted as the collapse pressure.
2.4.4
Flexibility/Bending stiffness
The bending stiffness is a measure of a structure’s resistance to bending deformation. It is the reciprocal of flexibility . The bending stiffness of the balloon section with mounted scaffold was measured using an experimental setup where the test sample is fixed at the free bending length l and deflected by the distance f . The resulting bending force F (load cell PW4MC3/300G-1, Hottinger Baldwin Messtechnik GmbH, Darmstadt, Germany, measurement range ± 3 N) is measured for small deflections. The force–distance curves describe the spring modulus of the test objects for bending. The bending stiffness EI is calculated taking the mean value of the slope F/f calculated by linear regression from the whole force–distance curve (beam theory). Considering possible asymmetric structures of the test samples, the bending stiffness was measured in five directions around the circumference and subsequently averaged. The bending stiffness was also measured for expanded scaffolds where a low stiffness is assumed to enable easy adaptation to vessel curvatures.
2.4.5
Statistics
Pooled variance t-test for independent samples (IBM SPSS Statistics 22, IBM Corp., Armonk, NY, USA) was used for statistical analysis. The significance level was set as p < 0.05.
3
Material
A total of three different balloon-expandable bioresorbable scaffolds for the treatment of coronary artery disease were included in the present study ( Fig. 3 ).
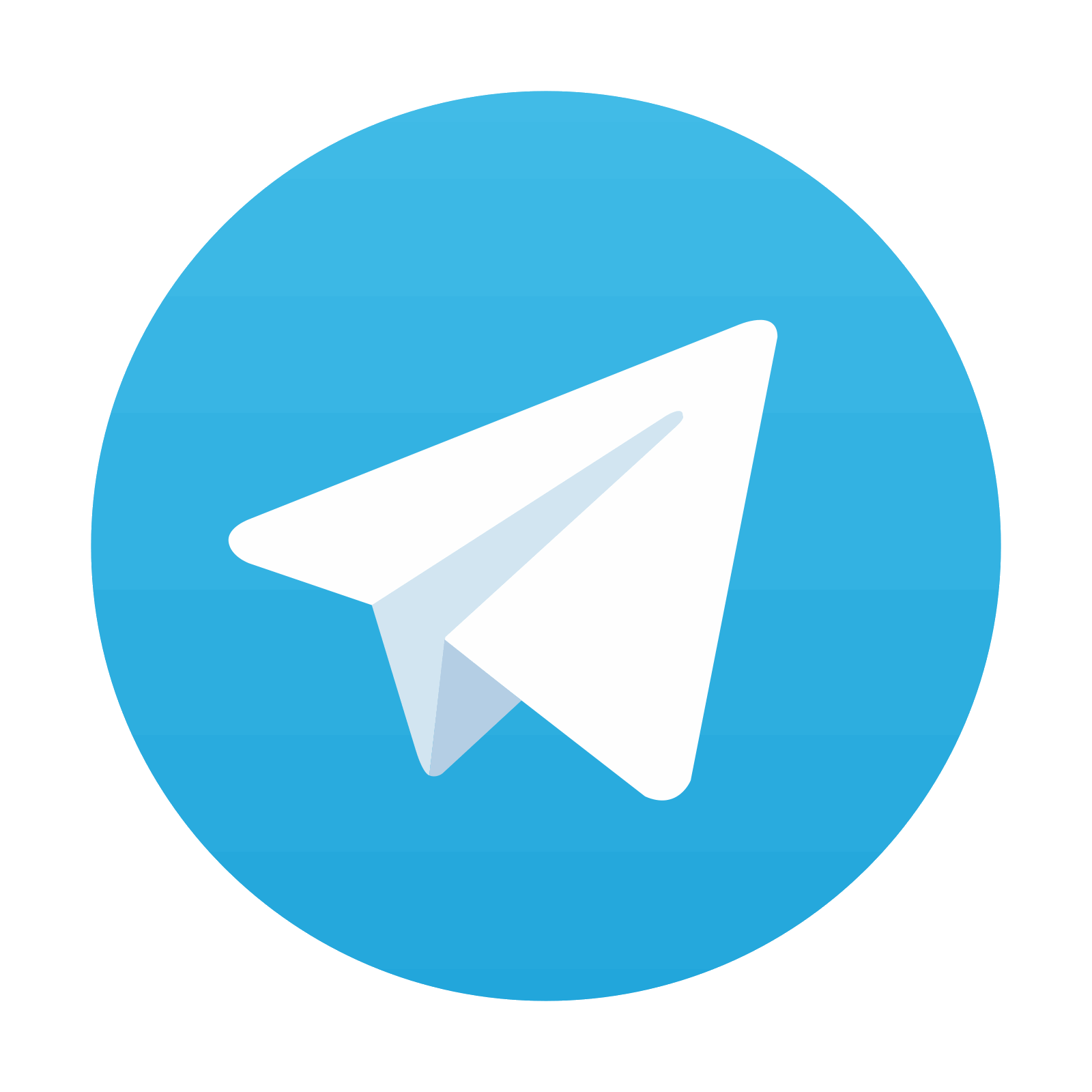
Stay updated, free articles. Join our Telegram channel
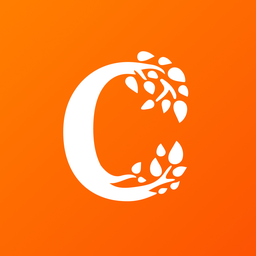
Full access? Get Clinical Tree
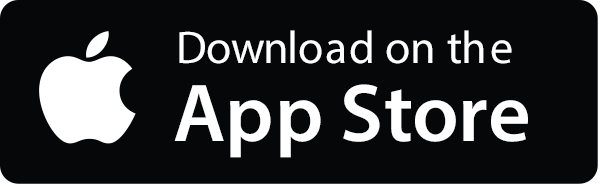
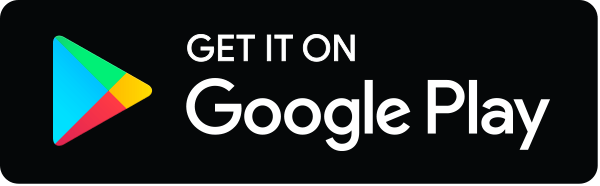
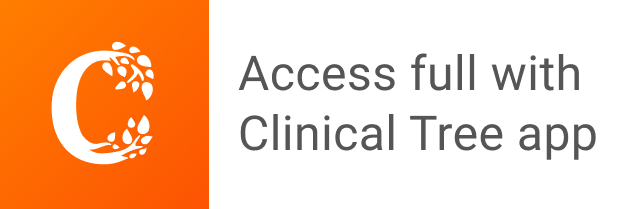