Fig. 20.1
Representation of several components of a single skinned cardiomyocyte setup (a) where a permeabilized (skinned) cardiomyocyte (b) is glued between a force transducer (FT) and a servomotor (SM) allowing assessment force and force-derived parameters under isometric conditions. A typical contraction-relaxation sequence is shown in (c). After transferring the myocyte from relaxing to activating solution (red line), isometric force starts to develop. Once a steady-state force level has been reached, the cell is shortened within 1 ms to 80 % of its original length (slack test) to determine the baseline of the force transducer. The distance between the baseline and the steady force level is the total force (Ftotal = Factive + Fpassive). After 20 ms, the cell is restretched and returns to the relaxing solution (blue line), in which a second slack test of 10-s duration is performed to determine resting or passive force (Fpassive). Using several Ca2+-containing solutions it is possible to extract and normalize the value of Factive to maximal Factive and construct a pCa versus force curve (d). From this graph is calculated the value of pCa50 (-log[Ca2+] for half-maximum force) and this parameter represents an index of myofilaments Ca2+ sensitivity
The experimental protocol usually consists of a series of force measurements upon Ca2+ stimuli (using Ca2+ buffer solutions with a pCa (− log[Ca2+]) ranging from 9.0 to 4.5), the determination of actin-myosin crossbridge kinetics and the measurement of the passive tension of the mounted cardiomyocytes for certain sarcomere lengths. For example, isolated rat cardiac cells have a length of ∼70–170 μm and diameters between 20 and 40 μm, often with irregular cross sections (Fig. 20.1b). They develop maximal isometric forces of ∼12 μN. The rate constant of force redevelopment following rapid release-restretch maneuvers is ∼5 s−1 after a length change of 20 % of the total cell length in 2.5 ms (Fig. 20.1c). Basic experiments, such as isometric force measurements, require a force sensor system that resolves forces below 0.1 μN, yet is sufficiently stiff so isometric conditions are maintained and sarcomere length is changed by less than ∼0.1 %. Over time, several force transducers have been developed for mechanical measurements on single cardiomyocytes such as capacitive force transducers, optical fibers, suction pipettes, glass needles, and microfabricated polysilicon beams as cantilevers [1, 2, 18, 19]. Force can be estimated either with an open loop system using a relatively non-compliant probe whose displacement is a measure of force [1, 2, 19] or a closed loop system in which a feedback control loop is used to stabilize the position of the probe, with the resulting control signal being a measure of force [12]. Cells are attached directly to the force transduces and the motor either by impalement, mechanical knots, wax or silicone glue, suction or polylysine [12].
A good force transducer is designed to comprise the following features: (1) high sensitivity with sufficient frequency response (resonant frequency around 250 Hz), (2) freedom to select any 3-D orientation of the transducer, (3) a simple and fast calibration procedure, (4) continuous analog signal, (5) little temperature drift and (6) good linearity and dynamic range [12]. Indeed, the transducer is the key element in the isolated skinned cardiomyocytes technique, enabling to measure several force and force related parameters produced by cardiac myofilaments. This system is well suited to evaluate contractility, stiffness and Ca2+ sensitivity baseline or upon stimulation with drugs acting directly on myofilaments. Many skinned cardiomyocytes apparatus allow attaching cells to a small amount of glue which is placed on the tip of micropipettes attached to a force transducer and to a length controller (Fig. 20.1b).
Most isolated skinned cardiomyocytes systems control and measure force and length, maximal Ca2+-activated force (Factive), stiffness (Fpassive), myofilaments Ca2+ sensitivity of isometric force (pCa50, using different Ca2+ containing solutions to construct pCa versus force curves, Fig. 20.1d), actin-myosin turnover rate assessed by isometric tension redevelopment rate (kTR), myofilaments cooperativity (nHill) and sarcomere length dependence of Factive, Fpassive, pCa50, nHill, kTR.
The advantages of this system include the possibility to: (1) use diminute amounts of frozen tissue (transported and stored at ≤−80 ºC) as a source of cardiomyocytes; (2) test a wide range of species, including mice, rats, rabbits, guineas pigs, pigs and humans; (3) reproduce physiological (e.g. stretch) and pathophysiological (e.g. oxidative stress) conditions in vitro; (4) conduct inexpensive experiments as pharmacological studies use very small amount of drugs or enzymes and (5) assume and rely on an uniform alignment of the myofibrils.
Other similar setups were developed prior to this one. Le Guennec et al. [20] presented a technique that allowed controlling preload of a cardiomyocyte fixed to carbon fibers. Thus it was possible to increase the length of the sarcomere homogeneously and calculate the passive and active forces by optically monitoring changes of the carbon fibers curvature under an auxotonic contraction. More recently, Nishimura et al. [21] modified Le Guennec technique presenting a system that controlled force and length. This method can be used in isolated cardiomyocytes during isometric, isotonic and auxotonic contractions. Two carbon fibers anchor the cell – one fiber is stiff, serving as a mechanical anchor, whereas the bending motion of the compliant fiber is monitored for force length measurement. Furthermore, by controlling the position of the compliant fiber using a piezoelectric translator, it is possible to change load dynamically during contractions. This apparatus allowed performing for the first time physiological force-length loops in isolated myocytes. A disadvantage of this technique is the absence of control of the position of cardiomyocytes thus biasing sarcomere length accurate assessment.
Basic physiology of cardiac muscle can also be studied at the level of the sarcomere. Cardiac myofilament activity, the ultimate determinant of cellular dynamics and force, is a central player in the integration and regulation of pathways crucial to cardiac function and can be assessed by atomic force microscopy. Most atomic force microscopy sensors utilize small silicon cantilever beams with dimensions in the range of several micrometers. They have high resonance frequencies (often >10 kHz) and high stiffness (∼10–1,000 N/m). With the appropriate detection system (i.e., laser beam deflection, tunneling current, interferometer), these sensors can reach the required nN resolution necessary for experiments on cardiac myocytes [18]. Despite these excellent properties, atomic force microscopy sensors are not well suited for measurements on cardiac myocytes. First, the diameters of single cardiac cells approach the dimensions of the cantilever of typical atomic force microscopy sensors [18]. It is therefore difficult to attach single myocytes exactly to the end of the beam and to accurately define the loading and the effective length of the cantilever. Second, the glue necessary to attach the cell must be distributed over a relatively large area of the beam such that its mechanical properties are changed. Third, atomic force microscopy sensors are fragile. Removal of glue residue and cell debris from the beam is not possible, and a new sensor is required for each cell [18].
20.2.2 Multicellular Preparations
In vitro studies have traditionally used multicellular preparations, such as papillary muscle or trabeculae, for the evaluation of cardiac mechanical properties including inotropic, lusitropic as well as basic electrophysiological and pharmacological properties. Actually, these preparations represent one of the oldest techniques used for the assessment of myocardial contractility and many fundamental concepts of muscle mechanics have been based on studies in this preparation, dating back more than 60 years [4]. Multicellular preparation data closely correlate with qualitatively results obtained from intact ventricle studies and thus become an invaluable tool to assess myocardial performance in conditions such as cardiomyopathy or heart failure. In these circumstances, parameters such as pressure and volume, which apply to the whole heart, are replaced by force or tension and length. By definition, force means the absolute amount of contractile strength, measured in newtons (N), whereas tension is force related to cross sectional area, measured in newtons per squared millimetre (N/mm2).
Protocols aimed to assess cardiac function in multicellular preparations typically involve muscle twitches (contraction–relaxation sequences) of either isotonic or afterloaded isometric contractions, but the frequency of contraction is very low compared to the physiological frequencies [22]. An isolated papillary muscle preparation responds to an electrical stimulus of suprathreshold intensity with an isotonic, an isometric or an auxotonic contraction (Fig. 20.2).
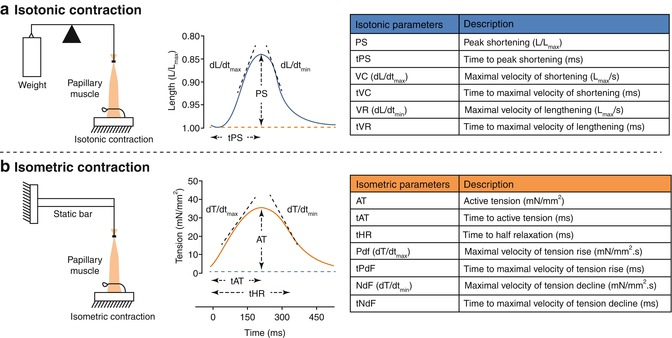
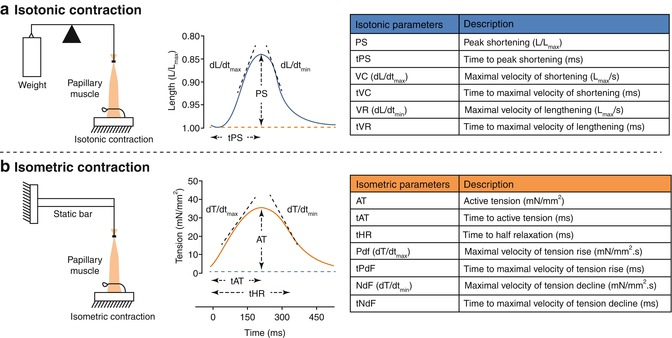
Fig. 20.2
Isotonic (a) and isometric (b) contraction in multicellular preparations. Left: different setups to assess mechanical properties of papillary muscles using isotonic or isometric contraction. In the isotonic contraction the preloaded muscle lifts the load/weight by shortening the contractile element without distension of the elastic component. In the isometric contraction the muscle develops tension by shortening the contractile element and thereby stretching the elastic component, with the overall length of the muscle remaining constant. Middle: the upper panel represents length of the muscle twitch over time while the lower panel represents tension of the muscle twitch over time. During an isotonic contraction (blue lines) the tension remains constant (blue dotted line in the lower panel) but the muscle length changes (blue full line in the upper panel). During an isometric contraction (orange lines) the length remains constant (orange dotted line in the upper panel) but the muscle tension changes (orange full line in the lower panel). Some parameters represented in these panels are: AT active tension, dL/dt max maximal velocity of shortening, dL/dt min maximal velocity of lengthening, dT/dt max maximal velocity of tension rise, dT/dt min maximal velocity of tension decline, PS peak shortening, tAT time to active tension, tHR time to half relaxation, tPS time to peak shortening. All velocity parameters are obtained by the first derivative of tension or length over time. Right: Isotonic and isometric contractions derived parameters and the respective units. All parameters are normalized to muscle cross sectional area
During contraction of a muscle in an isotonic setup, the muscle is fixed to the base, while the other end is attached to a loose transducer. Thus, when the muscle is electrically stimulated to contract under isotonic conditions there will be a displacement of the transducer and this movement is registered [23] (Fig. 20.2a).
During an isometric force measurement, force develops while shortening is prevented because the muscle is attached to two fixed ends one of which is a transducer. Upon electrical stimulation, the developed force is recorded under isometric conditions by the transducer. An important parameter to assess in such in vitro preparations is the Lmax – the length for which the muscle develops maximal force (Fig. 20.2b).
In these multicellular setups mechanical load, such as preload and afterload are controlled and influence the pattern of contraction of the muscles. Also quick changes in load and length have been used to give information about cardiac muscle mechanics. The preload is responsible for the initial muscle stretch and thus its length. After stimulation, the contractile elements of the muscle begin to shorten, but the length does not change: the muscle fibers shorten at the expense of the interaction of the myofilaments. The stretching of the elastic members results in a progressive increase of the force developed, but no muscle shortening. When the contractile force equals the load (afterload), the muscle shortens without increasing the developed force. The speed and force of contraction are dependent on the concentration of intracellular free Ca2+. Muscle force and speed are inversely related, so without load the speed is maximal but the force is residual. In contrast, in isometric contractions muscle does not shorten, its velocity of shortening is zero but develops maximal force [24].
These experiments are usually performed in organ bath heated to physiological temperatures containing oxygenated carbonate buffers (see Sect. 20.2.3.1). At the bottom of the bath there is a small device to oxygenate the solution or instead oxygen can be dissolved in the carbonate buffer that is perfusing the tissue. The pH of this solution should be strictly monitored and kept between 7.37 and 7.42 by altering the 95 % O2/5 % CO2 bubbling intensity. The base of the papillary muscle/trabeculae is fixed by a clamp, while the opposite end is attached to a transducer. In the organ bath there is a sensor that, together with a heater, controls the bath temperature [25]. The papillary muscles need to be stimulated electrically. Since the solution contains salts, it is possible that electrolytic processes occurring at the electrodes could lead to polarization and release of metals from the electrodes. This is usually prevented by using non polarizable electrodes such as platinum [25]. The buffer solution(s) chosen for the dissection and maintenance of the tissue is important as it will affect the viability of the preparation and hence the experimental protocol.
The basic procedure to obtain a stable preparation before starting any protocol typically includes the intravenous administration of anesthesia and heparin to the animal, to avoid the deposition of blood clots that potentially damage the endocardial endothelium. A thoracotomy is performed followed by the excision and fast immersion of the heart in the carbonate buffer. The heart is allowed to contract for a few beats to eject all the blood kept inside the ventricles coronary circulation and then is transferred to a similar solution but containing a cardioplegic agent to arrest the heart. Cardioplegia allows a safe dissection of the trabeculae or papillary muscles, which are subsequently transferred to the heated organ bath. The electric stimulation is turned on and the cardioplegic solution is replaced by normal carbonate buffer. During all this procedure the temperature should be kept as close as possible to the physiologic temperature of the animal.
Usually the derived parameters include active tension, maximum velocity of tension rise (dT/dtmax), maximum velocity of tension decline (dT/dtmin), peak shortening, time to peak shortening, maximum velocity of shortening (dL/dtmax), maximum velocity of lengthening (dL/dtmin) and time to half relaxation (Fig. 20.2). However other parameters can be derived from these, such as coefficients R1 and R2. Because changes in the contraction phase induce coordinated changes in the relaxation phase, variations in contraction and relaxation must be considered simultaneously to quantify drug induced changes in lusitropy [26]. Coefficient R1 = dL/dtmax/dL/dtmin evaluates the lusitropy under isotonic conditions where the amplitude of sarcomere shortening is greater than that observed under isometric conditions [27]. Because of the lower sensitivity of myofilament for calcium when cardiac muscle is markedly shortened under low load, relaxation proceeds more rapidly than contraction, apparently due to the rapid uptake of calcium by the sarcoplasmatic reticulum. Thus, R1 tests sarcoplasmic reticulum uptake function [28]. Coefficient R2 = dT/dtmax/dT/dtmin evaluates the lusitropy under a high load. When the muscle contracts isometrically, the sarcomeres shorten very little [27]. Because of the higher sensitivity of myofilament for calcium [29], the time course of relaxation is determined by calcium release from troponin C rather than by calcium sequestration by the sarcoplasmatic reticulum. Thus, R2 indirectly reflects myofilament calcium sensitivity [28].
Some of the advantages of the multicellular preparations include their great usefulness in elucidating the myocardial effects and mechanisms of action of certain drugs and neurohumoral agents while excluding the influence of systemic and other neurohumoral processes that occur in intact animals. Thus, they represent a classical pharmacological tool to assess dose response relationships in contractile tissue which is widely used in preclinical safety studies. Secondly, these preparations allow to measure and control force and length in preparations with relatively uniform fiber orientation, more than in the whole heart but less than in single cardiomyocytes, and to measure isotonic or isometric muscle performance independently of geometric constraints and interaction with blood flow and vascular tone. The results obtained from these organ bath systems are generally more consistent, reproducible and useful for measurement of concentration response curves. Finally, papillary muscles or trabeculae can be excised with minimal mechanical injury and remain stable for hours.
Some limitations of this preparation include the fact that: (1) the papillary muscle preparation has a higher elasticity than the one assumed due to end compliance, related to injured muscle extremities and the compliance of attached recording devices. This often results in significant mid-segmental shortening (up to 12 % Lmax) during supposedly isometric contractions [4]; (2) the resultant spatial inhomogeneity of sarcomere length and intracellular Ca2+ may invalidate many of the assumptions required for cardiac function assessment; (3) there is no simple relationship between active and passive elements in this preparation; (4) it is not possible to undertake voltage clamp studies, thus greatly diminishing its usefulness in studying excitation-contraction coupling processes [4]; (5) there is a potential inadequacy of oxygenation and substrate supply by the carbonate buffer to the inner parts of the muscles (hypoxic core) and (6) the accumulation of metabolites, such as inorganic phosphate, and concentration gradients in the organ bath [4]. In this regard, an appropriate selection of muscles with relatively low cross-sectional areas (<0.5 mm thick) is critical and may surpass these limitations.
Regarding the influence of endothelial cells on myocardial function, it is important to account for the important functional differences between endocardial and coronary vascular endothelial cells [30]. Moreover, the coronary vascular endothelial cells, unless perfused, are probably non-functional in this preparation [31] in contrast to the endocardial endothelial cells whose interaction with the myocardium has been quite well studied in the papillary muscle [30]. In addition, important and relevant aspects of endothelial function, such as cyclical mechanotransduction mediated by shear stress are largely inoperative.
Despite these limitations, a number of recent technical breakthroughs provide the potential to overcome some of these problems. Among the most important of these is the ability to accurately assess sarcomere length, as well as, to obtain true isosarcometric contractions using laser diffraction to monitor sarcomere length and adaptive control systems to regulate it [32]. Interestingly, this approach demonstrated that many “isometric” conditions assumed in previous studies may not be totally reliable. Laser diffraction analysis coupled with force measurements provides important information about muscle contractile properties. Moreover, the incorporation of objective measurement of variables such as intracellular Ca2+ transients, ATPase activity, heat production and O2 consumption [32, 33] are a major advance over much less precise and speculative interpretative assessments based solely on mechanical correlates. Other progresses include the use of skinned fibres with careful, uniform control of Ca2+ levels for studying Ca2+-myofilament interaction, and the use of caged compounds [34]. In addition, information about the availability of stored calcium to activate muscle contraction is studied using rapid cooling contracture techniques. Rapid cooling contractures are also useful for assessing sarcoplasmic reticulum Ca2+, especially in multicellular preparations where slow caffeine diffusion to all the cells limits the utility of the caffeine approach. Cooling to 0 °C inhibits Ca2+ pumping and also causes rapid sarcoplasmic reticulum Ca2+ release (presumably due to very long ryanodine receptor openings). Then, one can measure either Δ[Ca2+]i or contractile force (which develops slowly at 0 °C). This technique is less quantitative concerning absolute amounts of Ca2+ but is useful for measuring changes in sarcoplasmatic reticulum Ca2+ content under different conditions.
20.2.3 The Isolated Perfused Heart
The ex vivo isolated perfused heart includes two different preparations: the Langendorff preparation, first described by Oskar Langendorff in 1895 [35] who demonstrated that the heart receives its nutrients and oxygen from blood via the coronary arteries and that cardiac mechanical function is reflected by changes in the coronary circulation and the ejecting heart or “working heart” system developed by Howard Morgan and James Neeley in 1967 to investigate the metabolism of the heart and coronary regulation [36].
20.2.3.1 Langendorff Preparation
In the Langendorff preparation, blood or more commonly crystalloid perfusates (nutrient solution), are delivered into the heart through a cannula inserted in the ascending aorta, either at constant pressure or constant flow. Retrograde (reverse) flow in the aorta closes the aortic valve and, as a result, the entire perfusate enters the coronary arteries via the ostia, located just outside the valve at the aortic root, thus maintaining the viability of the heart muscle. After passing through the coronary circulation the perfusate drains into the right atrium via the coronary sinus and tends to drip from the apex of the heart making it easily available for collection (Fig. 20.3a). This preparation is useful for cardiomyocyte isolation as well as for physiological monitoring of cardiac function using a system where stable preparations perfusion of several hours is usual.
< div class='tao-gold-member'>
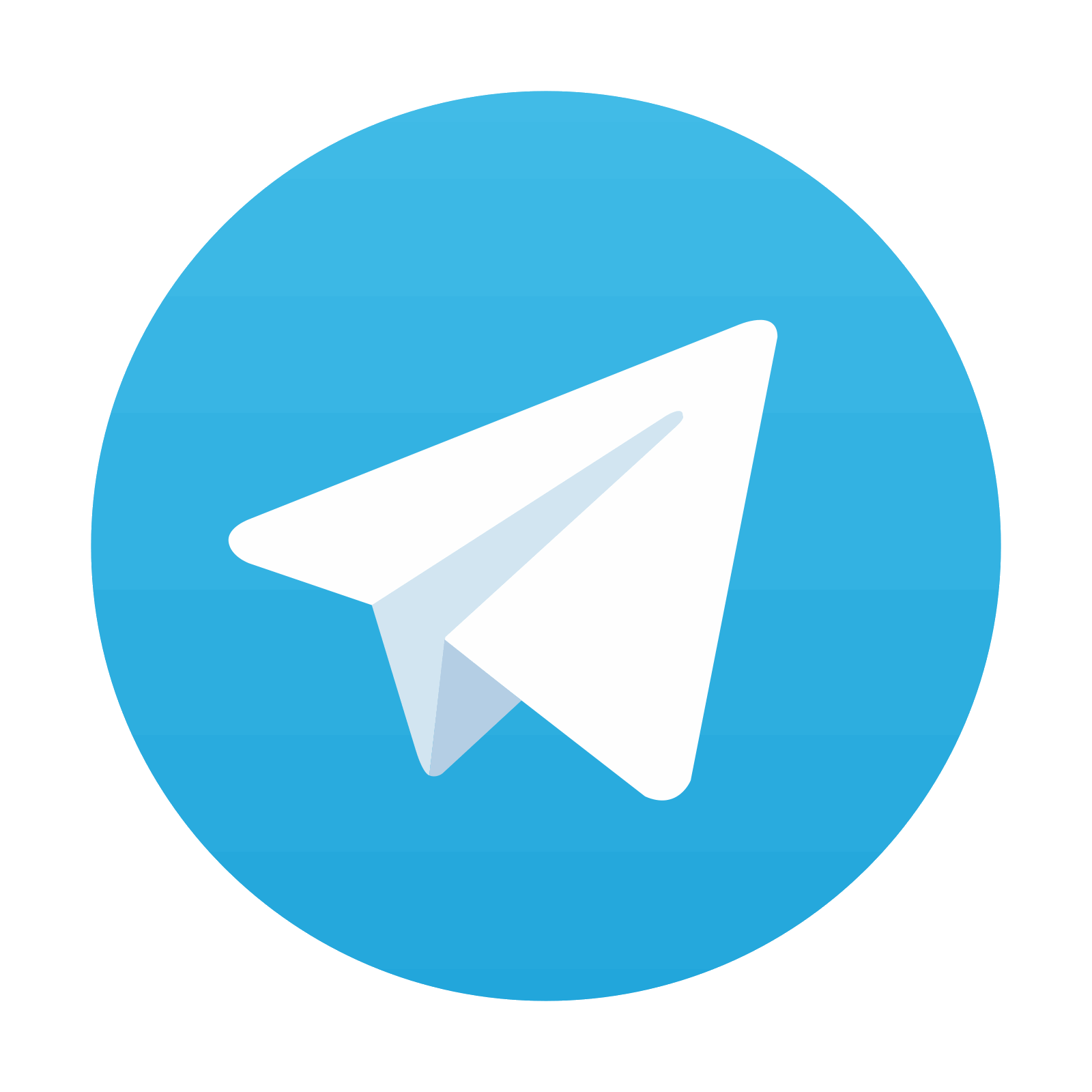
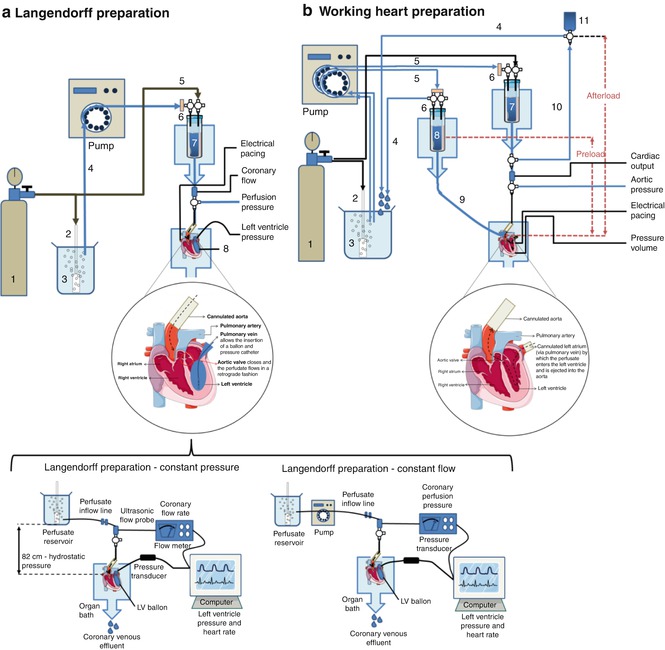
Only gold members can continue reading. Log In or Register a > to continue
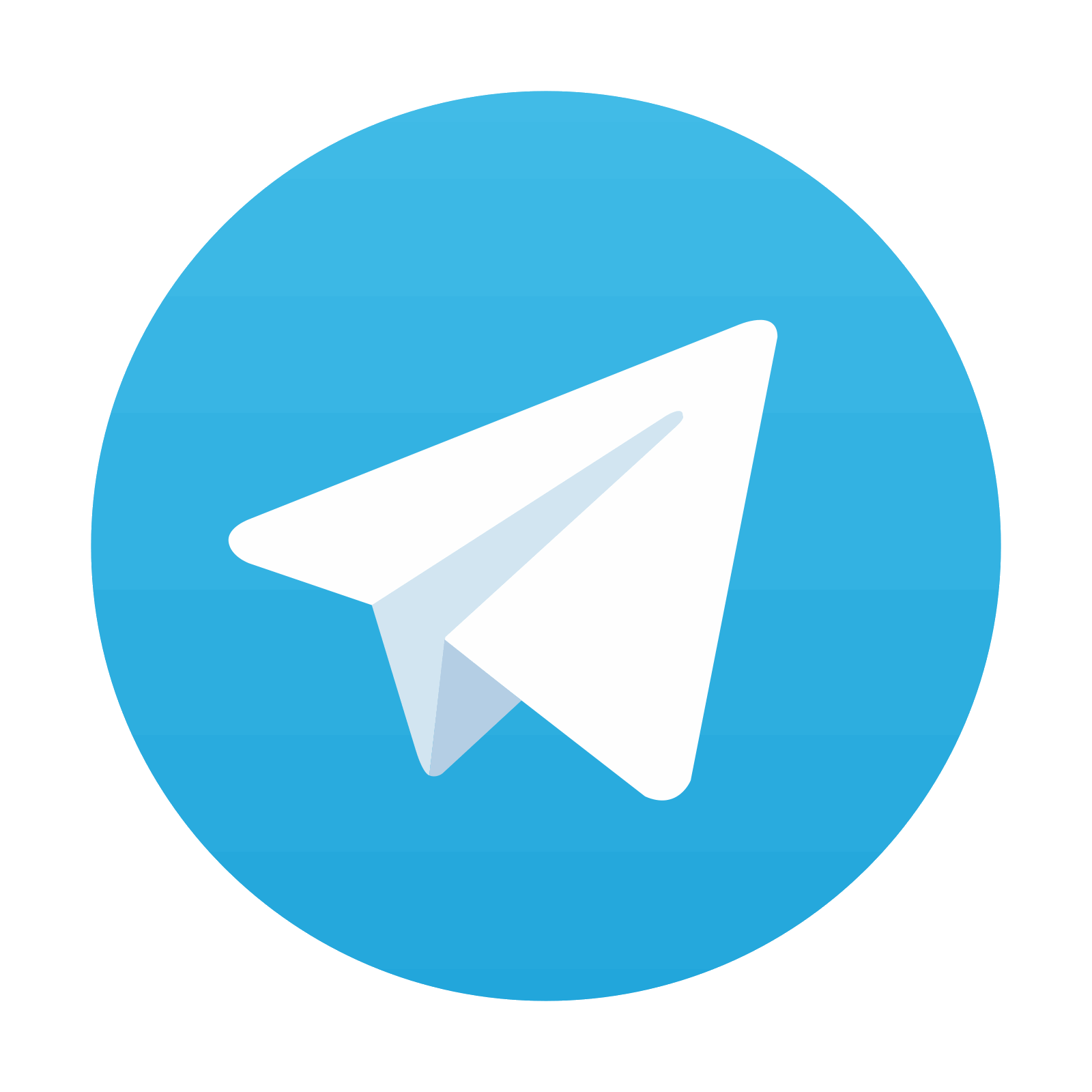
Stay updated, free articles. Join our Telegram channel
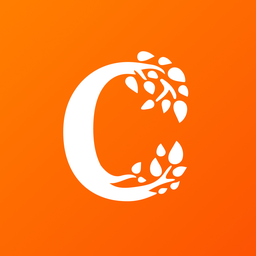
Full access? Get Clinical Tree
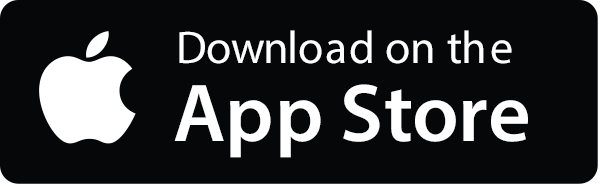
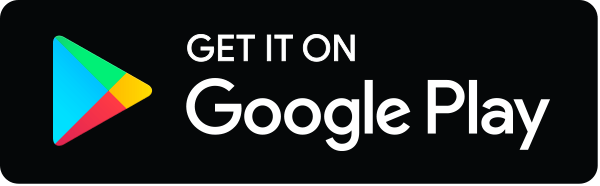