LEARNING OBJECTIVES
Learning Objectives
The student will be able to use the Fick equation to calculate O2 consumption and aerobic capacity, using proper units and citing ranges for average levels.
The student will be able to explain the relationship between pulmonary gas exchange and exercise intensity, and the effects of endurance training.
The student will be able to summarize the effects on aerobic capacity of genetics, normal aging, bed rest, and detraining.
A subject’s aerobic capacity is an important assessment in respiratory physiology because the lungs may comprise a primary limitation on O2 transport and V̇o2 (Chap. 1). Furthermore, abundant scientific evidence supports the contention that aerobic capacity is a very powerful predictor of both life expectancy and mortality from many diseases. Aerobic capacity has several synonyms in the literature, including exercise capacity, aerobic fitness, maximal or peak oxygen consumption (V̇o2max and V̇o2peak, respectively), metabolic equivalent of the task (MET) capacity (see below), and cardiovascular fitness. For consistency, the terms aerobic capacity and V̇o2max will be used throughout this chapter.
DEFINITION AND CALCULATION OF AEROBIC CAPACITY
Aerobic capacity is defined by the American College of Sports Medicine (ACSM) as “The ability to perform dynamic exercise that involves large muscle groups at a moderate to high intensity for prolonged periods.” V̇o2max is the most widely accepted measure of such aerobic capacity. Recall that V̇o2 always is equal to the product of the amount of blood delivered to all body tissue, that is, the cardiac output, multiplied by the amount of oxygen extracted from the blood during each passage through the tissues, that is, its arterial-venous oxygen content difference. Mathematically this relationship is expressed by the Fick equation:
Clearly an individual’s V̇o2max may be limited by any factor that constrains either cardiac output or the (arterial-venous) O2 content difference. Each of these components will be discussed in this chapter. One general comment is worth noting at this point. When evaluating aerobic capacity using weight-bearing exercises like running, walking, or climbing stairs, the V̇o2max is expressed in mL/kg/min or L/kg/min to correct for differences in a subject’s body weight (and from which can be calculated their body mass index, BMI). When evaluating aerobic capacity using non-weight-bearing exercises like swimming, rowing, or biking, then V̇o2max is expressed in mL/min or L/min to exclude influences of a subject’s body weight.
A useful alternative is to express V̇o2max in METs, with one MET being the average for a subject’s resting V̇o2. Historically, 1 MET = 3.5 mL/kg/min, although recent research indicates that a truer estimate over a wider range of body weights is 2.6 mL/kg/min. Thus, a subject with V̇o2max of 8 METs has an aerobic capacity approximately seven-fold above their resting metabolic rate. This subject would also be described as having a metabolic reserve of 7 METs. Tables 12.1 and 12.2 provide normative data for weight-adjusted aerobic capacity (mL/kg/min) for subjects sorted by age and sex. The verbal classification rankings in each table are useful in distinguishing among patients from a range of lifestyles, and have been validated using the ACSM Guidelines Handbook.
Age (y) | 20-29 | 30-39 | 40-49 | 50-59 | 60-69 | 70-79 | Classification |
---|---|---|---|---|---|---|---|
95% | 56.2 | 54.3 | 52.9 | 49.7 | 46.1 | 42.4 | Superior |
80% | 51.1 | 47.5 | 46.8 | 43.3 | 39.5 | 36.0 | Excellent |
60% | 45.7 | 44.4 | 42.4 | 38.3 | 35.0 | 30.9 | Good |
50% | 43.9 | 42.4 | 40.4 | 36.7 | 33.1 | 29.1 | Fair |
40% | 42.2 | 41.0 | 38.4 | 35.2 | 31.4 | 28.0 | Fair |
20% | 38.1 | 36.7 | 34.6 | 31.1 | 27.4 | 23.7 | Poor |
1% | 26.6 | 26.6 | 25.1 | 21.3 | 18.6 | 17.9 | Very poor |
Age (y) | 20-29 | 30-39 | 40-49 | 50-59 | 60-69 | 70-79 | Classification |
---|---|---|---|---|---|---|---|
95% | 50.2 | 46.9 | 45.2 | 39.9 | 36.9 | 36.7 | Superior |
80% | 44.0 | 41.0 | 38.9 | 35.2 | 32.3 | 30.2 | Excellent |
60% | 39.5 | 36.7 | 35.1 | 31.4 | 29.1 | 26.6 | Good |
50% | 37.4 | 35.2 | 33.3 | 30.2 | 27.5 | 25.1 | Fair |
40% | 35.5 | 33.8 | 31.6 | 28.7 | 26.6 | 23.8 | Fair |
20% | 31.6 | 29.9 | 28.0 | 25.5 | 23.7 | 21.2 | Poor |
1% | 22.6 | 22.7 | 20.8 | 19.3 | 18.1 | 16.4 | Very poor |
INFLUENCE OF AEROBIC CAPACITY ON MORTALITY
Aerobic capacity is a more powerful predictor of mortality than any other established risk factor for cardiovascular disease. Indeed, as reported in the New England Journal of Medicine in 2002 by Myers and colleagues, a high aerobic capacity is protective even in the presence of other known risk factors (Fig. 12.1). When subjects are defined according to other known risk factors like diabetes, the risk of death from any cause in subjects whose exercise capacity is <5 METs is nearly double that of subjects whose exercise capacity is >8 METs.
FIGURE 12.1
Relative risk of death by any cause in subjects with various risk factors who achieved aerobic capacities of <5 METs, 5-8 METs, or >8 METs. Data are based on 6213 patients of mean age = 59 years. From Myers et al: Exercise capacity and mortality among men referred for exercise testing, N Eng J Med Mar 14; 346(11):793-801, 2002.
Because physical fitness is a modifiable risk factor, improving it enhances patient prognoses: Each MET of increased aerobic capacity yields ~12% improvement in survival. As shown in Fig. 12.2, mortality rates differ strikingly just between the least-fit quintile (lowest 20% of the population) and the next-least fit quintile (the second-lowest 20% of the population). Regardless of whether patients have cardiovascular disease, survival declines as aerobic capacity decreases. In this multicenter study, fewer than 50% of patients with an aerobic capacity <5 METs survived 14 years from the study’s inception versus >75% of patients with an aerobic capacity >8 METs.
FIGURE 12.2
Age-adjusted risk of death from any cause by quintiles of exercise capacity in normal subjects or patients with cardiovascular disease. Subgroups with the highest exercise capacity (quintile #5) are the reference cohort in each. From Myers et al: Exercise capacity and mortality among men referred for exercise testing, N Eng J Med Mar 14; 346(11): 793-801, 2002.
USING THE FICK EQUATION TO ESTIMATE V̇o2 AND V̇o2max
In 1870, the German physician and physiologist Adolf Eugen Fick developed the eponymous equation that was introduced above to estimate Q̇ when the O2 concentrations of arterial and venous blood and of the exhaled gas are known or can be measured. Thus, the Fick equation demonstrates the combined efficacies of the heart, lungs, and peripheral muscles to achieve a maximal effect systemically:
As introduced in Chap. 1, O2 delivery is defined from this equation as the product of Q̇ and Cao2, while O2 extraction is similarly defined as the difference between Cao2 and Cv̄o2. Therefore, V̇o2 equals the product of Q̇ and O2 extraction, and V̇o2max is the physiological optimization of all three terms by the heart (Q̇), the lungs (Cao2), and the working muscles (Cv̄o2). In determining V̇o2max for a healthy subject or a particular patient, the physician is often simultaneously assessing which of these three terms is rate-limiting under the clinical and environmental conditions present during assessment.
As established in Chaps. 3 and 9, the Cao2 depends on four variables: blood [Hb]; the binding capacity of Hb for O2 (1.39 mL O2/g Hb); the effective PAo2; and the amount of dissolved O2, also dependent on Pao2 (Chap. 3). Mathematically this becomes:
In a healthy nonsmoking man with [Hb] = 15.6 g/dL breathing air at sea level, this yields:
Anemia and methemoglobinemia reduce the effective arterial [Hb] and thus the maximal Cao2, while high altitude, hypoventilation, and pulmonary fibrosis reduce Cao2 by their effects on PAo2. Not surprisingly, physicians as well as many professional athletes are personally aware of training approaches and pharmaceutical agents that affect aerobic capacity by their impact on Cao2 (see Chap. 13). It is important for both clinical and lay audiences to remember that increasing Cao2 may not in itself increase aerobic capacity. For example, the polycythemia from administering erythropoietin may increase blood viscosity to the point where Q̇max
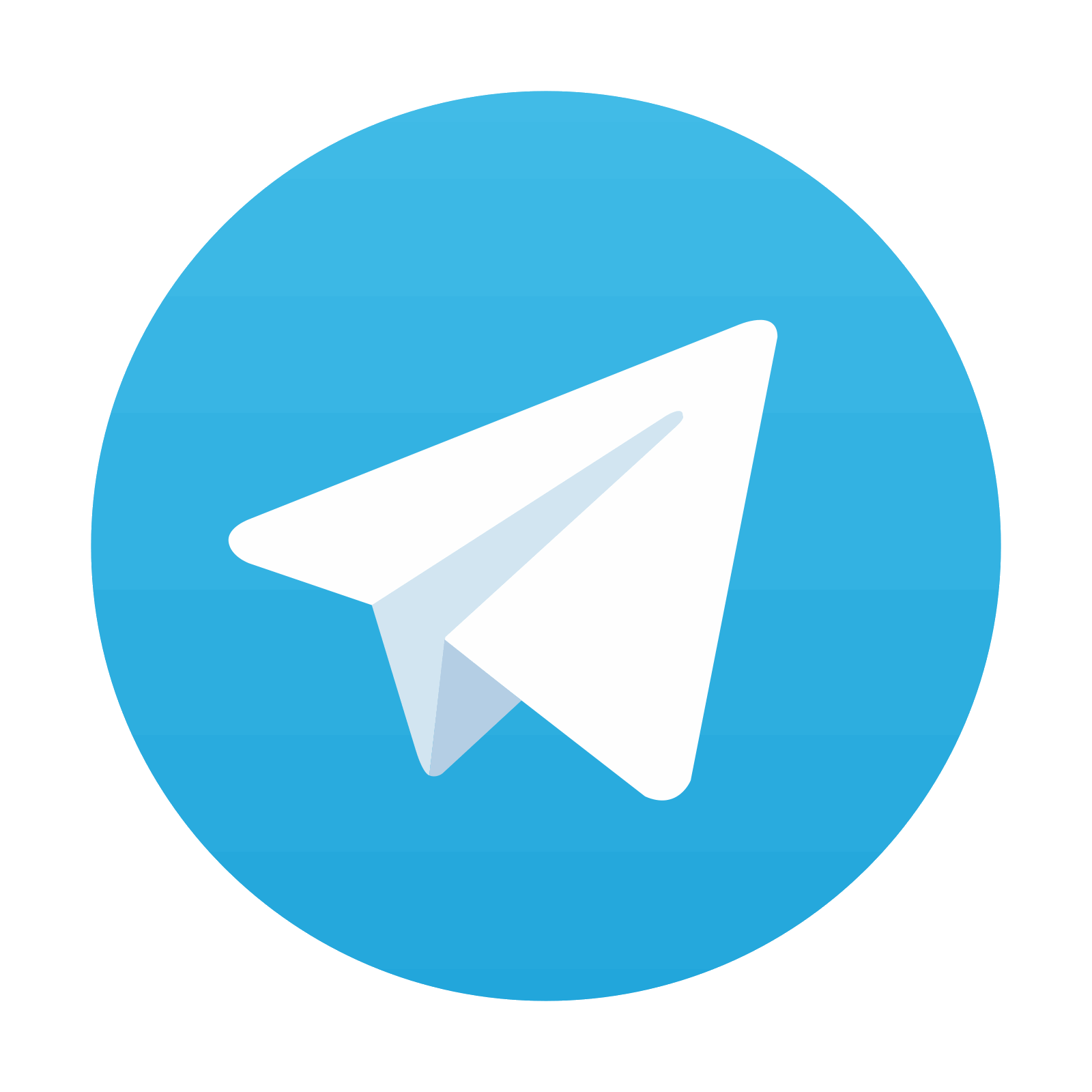
Stay updated, free articles. Join our Telegram channel
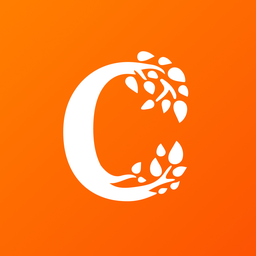
Full access? Get Clinical Tree
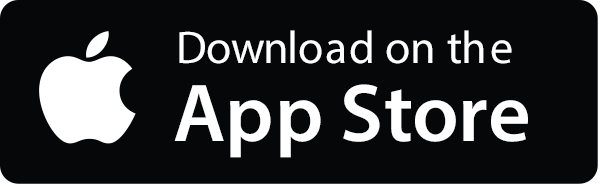
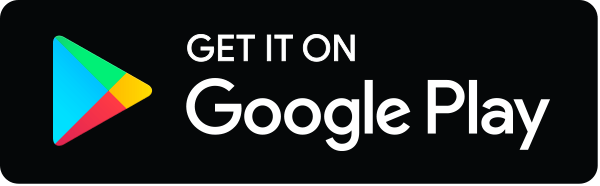
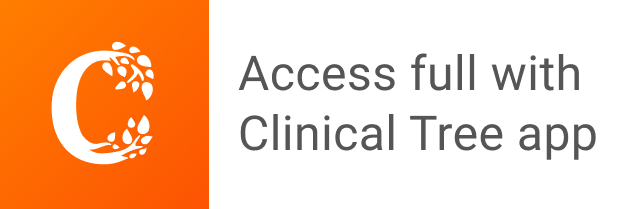