For over 40 years, cardiac and lung transplantation have achieved remarkable one-year patient survivals beyond 90% and conditional half-lives of 13 and 7.9 years, respectively.1 While surgeons must acquire the technical expertise to perform these often demanding surgeries, long-term graft outcomes and the recipients’ well-being benefit from a multidisciplinary team well versed in the basics of the immunology of transplantation. Comfort with treating patients who receive immunosuppressive therapies, both conventional and innovative, requires familiarity with the nonsurgical language of transplantation. The goal of this enhanced chapter is to squeeze the essentials into an understandable short text. The core features of the alloresponse are presented with some specific references unique to heart and lung allografts. These include (1) histocompatibility; (2) activation of alloresponse T lymphocytes; (3) T-cell–mediated rejection (TMR); (4) antibody-mediated rejection (AMR); (5) immunosuppressive therapy; (6) surveillance for rejection; (7) immune monitoring; and (8) emerging regulators of immunity.
Major histocompatibility complex (MHC) molecules are a family of proteins that vary quite a lot between individuals (genetic polymorphism) and represent the molecular basis for how people’s immune systems distinguish “self” from “nonself” with respect to infections and transplants. Human MHC molecules are known as human leukocyte antigens (HLA) because they are expressed at high levels on leukocytes and were first measured on peripheral blood lymphocytes. HLA are heterodimeric glycoproteins expressed on the surface of almost every cell in the human body. If recognized by an organ recipient, these proteins can trigger rejection. This is known as allorecognition.
HLA molecules on donor cells or HLA fragments, shed from thoracic organ transplants, are determined to be foreign by the immune system of the host. Intact HLA molecules expressed on the surface of cells serve two key functions in the context of transplantation. Fragments of foreign proteins, including fragments of HLA molecules, are presented in the binding groove of HLA and are recognized by T-cell receptors (TCRs) of the recipient that happen to have high affinity for that protein fragment in the context of self HLA (indirect donor antigen presentation). In addition, recipient T-cells directly recognize donor HLA as “foreign” (direct donor antigen presentation).
The genes coding for these antigens are located on the short arm of chromosome 6. This region spans over 4 million base pairs in length and encodes for over 200 genes in three regions: Class I, Class II, and Class III (Fig. 59-1). HLA owns the most polymorphic title in man as more than 5000 types have been recognized.
Classic Class I HLA proteins/antigens are HLA-A, HLA-B, and more recently HLA-C. The α-light chain is encoded in the MHC; however, the β-chain is β-2-microglobulin that is encoded on chromosome 15. When folded, the α-heavy chain contains the peptide binding region which presents peptide antigen to the T-cell. Class I antigens are constitutively expressed on nearly all cells. Expression of Class I molecules (Fig. 59-2) is upregulated on the endothelium and parenchymal cells in association with inflammation, including after ischemia and reperfusion.
FIGURE 59-2
Class I and II HLA molecules are made up of polypeptidic chains with intrachain disulfide bonds. The α1 and α2 distal domains of Class I and the α1 and β1 domains of Class II make up the peptide binding site for alloantigen. (Adapted with permission from Haber E: Scientific American: Introduction To Molecular Cardiology. Philadelphia; Elsevier Health Sciences; 1995.)

Classic Class II HLA proteins/antigens are HLA-DR, HLA-DRw, HLA-DQ, and more recently HLA-DP. These proteins consist of an α-heavy chain and a β-light chain, both of which are encoded in the MHC. When folded, the α-heavy chain and β-light chain come together with each contributing part of the peptide binding region (Fig. 59-2). Class II antigens are expressed mainly on B-cell lymphocytes, activated T-cells, monocytes/macrophages, and dendritic cells. Other cell types, such as endothelial cells, may express Class II antigen expression after activation.
Each person has two different HLA genes at each HLA locus (HLA-A, B, D, etc.) HLA antigens are co-dominantly expressed on the cell surface so that each cell has two different HLA proteins for each HLA locus. HLA “haplotype” (the sequence of A, B, D genes on one chromosome) is usually inherited as a group from each parent in a Mendelian fashion. Thus, two offspring of the same parents have a 50% chance of inheriting one identical haplotype, 25% chance of inheriting two identical haplotypes, and 25% chance of having no haplotypes in common. In addition, due to the distance between these genes in the MHC, there are “hot spots” of gene recombination. Recombination in offspring occurs approximately 1 to 2% between HLA-A and C, or between HLA-B and HLA-DR, and approximately 30% between HLA-DP and HLA-DQ. Recombination accounts for most of the occasional exceptions to faithful transmission of intact parental haplotypes to their children. HLA haplotypes are found in varying frequencies across the major population groups (White, Hispanic, Black, Asian, and American Indian).
In the early days of organ transplantation, hyperacute rejection occurred due to preexisting IgM alloantibodies. Best-known examples of these are the ABO blood group IgM antibodies. While still a barrier to xenotransplantation (cross-species transplantation), blood group typing has virtually eliminated this particular cause of immediate organ destruction in allotransplantation. Of interest is the success of blood group mismatched organs in neonatal recipients before they develop IgM antibodies to blood group antigens.2 Antibodies against blood group antigens arise between 6 and 18 months postnatal due to the presence of related carbohydrate antigens on intestinal bacteria. Today, when hyperacute rejection is seen, it is normally caused by preexisting IgG antibodies usually directed against donor HLA proteins. These antibodies are often the result of previous blood-cell transfusions (most particularly multidonor platelets due to the high HLA load) or previous pregnancies or transplants. After wide adoption of the first crossmatch technique by Patel and Terasaki in 1969, hyperacute rejection based on preexisting HLA antibodies became rare.3 Further improvements in screening for HLA antibodies have nearly eliminated it. Of interest in 1970, against early convention, Terasaki found that HLA-mismatched kidney recipients fared as well as those zero-mismatched recipients.4 Starzl led the use of mismatched livers first, and soon, except for bone marrow, all followed in kidney, heart, and lung transplants.5
While HLA mismatches seem less predictive of outcomes, those recipients who present for transplant with preformed anti-HLA antibodies (prior pregnancies or blood transfusions) or developed antibodies in responses to the mismatches have more rejection. This has led to poor survival in heart and lung recipients.6-10 There is some evidence that posttransplant removal of these donor-specific antibodies (DSA) can improve the function of kidneys and lungs.11,12
Success in heart and lung transplantation requires control over the immune response to donor HLA antigens expressed by the allograft. An adaptive response is initiated by T lymphocytes primed to donor antigens in the peripheral lymphoid tissues and are then recruited into the donor organ where those antigens are expressed. Alloreactive T lymphocytes are primed by alloantigens presented to them on donor (direct) and/or host (indirect) antigen-presenting cells (APCs) (Fig. 59-3). T-cells are the primary immune actors in the pathologic reaction to a transplanted organ. They participate in cytotoxicity and cytokine-mediated inflammation. B cells, antibodies, and macrophages contribute to the destruction of the graft via a variety of effector pathways. Ischemia/reperfusion injury of the allograft triggers innate immunity that can amplify T-cell alloresponse. Molecular modulators of innate immunity have recently been an expanding area of interest. These include cytokines, chemokines, complement, and more recently toll-like receptors (TLRs) and the inflammasome. While APCs are emphasized, B cells and natural killer (NK) cells exert influence on a host’s decision to reject an allograft.13
A T-cell can only become triggered to proliferate and differentiate if its TCR finds a fit with foreign peptides bound in the groove of HLA molecules presented on the surface of APCs.14 “Professional” APCs differ from other HLA-expressing cells in that they also express “co-stimulatory” molecules that efficiently stimulate activation of both the APC and the responding T-cell. These include dendritic cells, B lymphocytes, and macrophages. The peptide HLA antigen complex may be presented by resident donor “passenger” APCs found in the transplanted heart or lung (direct presentation) or by the host’s APCs (indirect presentation) (Fig. 59-4). HLA-peptide antigens become the specific key that fits into a T-cell’s specific TCR receptor. In the thymus, T-cells learn to enable their TCR to recognize self-HLA complexes that present endogenous peptides (positive selection). Those cells that recognize self-peptides with a high affinity are deleted (negative selection) so as to reduce the risk of their driving autoimmunity. T-cells that cannot bind self-protein HLA die from neglect. Positive selection occurs only for those T-cells whose affinity for self-protein HLA result in survival but not full proliferation. The T-cell repertoire emerging from the thymus can respond to proteins from various foreign origins (bacteria, viral, transplant). T-cells react against alloantigens because of the crossreactivity of the TCR with self and foreign HLA molecules. Host and passenger donor dendritic cells are thought to be the most efficient type of APCs for activating naive T-cells.
Donor passenger APCs transplanted with the heart or lung directly process endogenous HLA-derived peptides from within themselves and present them in a groove on surface Class I HLA complexes on their surfaces (Fig. 59-5A). Class I protein MHC complexes presented on the donor-derived APCs (key) fit into the TCR (lock) expressed by recipient CD8+ T-lymphocytes. This activation pathway, known as the direct pathway of peptide presentation, is responsible for the majority of initial T-cell activation reacting to alloantigens and thought to mediate most early events of acute cell-mediated cytotoxicity.15
FIGURE 59-5A
Direct presentation of Class I HLA donor antigen by donor APCs. Donor-derived APCs (dendritic cells within the transplant) ubiquitinate an endogenous cytosolic (donor) protein and transport it through the proteasome where it is digested. Small proteins move with transporter associated with antigen processing (TAP) into the endoplasmic reticulum where it becomes associated with Class I HLA α-chain. The protein HLA complex locates on the surface of the APC where CD8+ T-cells with TCR “locks” specific for the protein-HLA complex “keys” can associate. The association prompts CD8+ T-cell activation.

FIGURE 59-5B
Indirect Class II HLA donor-antigen presentation by host APCs. Extracellular protein antigen shed from the heart or lung allograft is taken into a host APC by endocytosis. The protein is proteolyzed and transported into the Golgi. There it displaces the invariant chain held α and β-chain Class II HLA molecule. The digested protein molecule finds its specific place within the HLA molecule and is moved to the APC surface where it can specifically adhere to a CD4+ T-cell. The attached CD4+ T-cell with donor-specific TCR is stimulated to proliferate and participate in the alloresponse.

Later alloresponses are focused on CD4+ T-cells because they recognize donor-specific HLA peptides presented by Class II surface molecules expressed by recipient APCs. These recipient APCs gradually replace donor APCs transplanted with the heart or lungs of the donor. Host APCs trafficking through the allograft phagocytose interstitial and cellular HLA protein antigens shed from the donor graft (Fig. 59-5B). This shedding is induced when the graft is stressed by ischemia (preservation), inflammation (bacterial and viral infection), and during rejection. Donor proteins undergo endosomic proteolysis and resulting peptides are combined with Class II HLA molecules. Donor peptide Class II HLA complexes are transported to the surface of the host APC and presented indirectly as the “key” to the TCR complex “lock” on CD4+ T-cells. These T-cells then become activated by the indirect pathway. Because CD4+ T-cells provide help to donor-specific B cells, later alloresponses tend to be associated with appearance of alloantibody.16
In addition to the direct and indirect pathways, a third pathway of antigen presentation involves the transfer of donor cell membrane components, including donor peptide-MHC complexes, to recipient APCs. Transfer can happen by direct cell-cell contact or through the release of very small particles (exosomes) by donor cells, which fuse with recipient APC cell membranes. This process known as the semi-direct pathway of antigen presentation pathway leads to presentation, by recipient APCs, of the same strongly crossreactive donor MHC/peptide that drive direct presentation.17 Finally, it is helpful to distinguish the role of these pathways in the priming versus the effector phase of immune responses.18
Activated dendritic cells also provide co-stimulators to the naïve T-cells that are required for a full T-cell response. Macrophages present antigens to differentiated CD4+ cells that activate the macrophage to promote cell-mediated immunity. B cells also serve an APC function by presenting antigens to helper T-cells. These then activate the B cell to become part of the effector humoral immune response through production of antibodies (Fig. 59-6).19
FIGURE 59-6
CD4+ T lymphocytes are specifically activated by donor peptide proteins presented on APC peptide-HLA complexes. These T-cells in turn activate B lymphocytes expressing specific HLA Class II molecules and CD40. The B cells respond to growth factors by proliferation formation of germinal centers and production of specific alloantibody.

In addition to activation of the T-cell by engagement of its TCR with donor peptide/MHC complexes expressed by donor or recipient APCs (Signal 1), several other surface molecules participate in T-cell activation. Those that enhance TCR signaling (CD4 and CD8 molecules expressed by CD4+ and CD8+ T-cells, respectively) are called co-receptors because they bind to part of the same HLA molecule that engages the TCR. Another group of T-cell activating surface molecules is called co-stimulatory receptors (Signal 2) (Fig. 59-7). Blockade of co-stimulatory pathways provides important new possible therapy to prolong organ transplant survival and even reach a tolerant state. Co-stimulatory receptors or ligands on T-cells recognize respective cognitive ligands or receptors presented on APCs or the graft tissue itself. The best defined co-stimulatory receptor and ligand pair is the constitutively expressed CD28 T-cell co-stimulatory receptor and its APC ligands B7-1 (CD80) and B7-2 (CD86). This pathway is particularly important in activation of naïve (nonmemory) T-cells. When engaged, these pairs deliver antiapoptotic signals and trigger the expression of cytokines and growth factors, including Interleukin-2 (IL-2) that encourage proliferation of alloantigen-specific CD4+ or CD8+ T-cells. If CD28 is blocked, T-cells may become anergic or apoptose. A second inducible co-stimulatory receptor for B7 molecules has been identified and termed CTLA-4 (CD152). Unlike the structurally homologous CD28, CTLA-4 appears on recently activated T-cells and is a negative regulator that promotes pathways that limit proliferation. It is efficient that the same co-stimulator (B7) can prompt initial proliferative signals via constitutive CD28 engagement and later, when CTLA-4 is induced, subsequently limit signals to the T-cell. Therapeutic targeting of this pathway with CD80 and CD86 blockade with CTLA-4-Ig-like molecules has been effective in primates and multiple clinical trials,20 and was approved by the US Food and Drug Administration (FDA) for the prevention of acute rejection in adult kidney transplant patients in 2011. Inducible co-stimulator (ICOS) and programmed death-1 (PD-1) are more recent discoveries in the T-cell CD28 family. When ICOS joins its ligand ICOS-L on an activated APC, it stimulates the T-cell effector response by promoting IL-4 and IL-10 production. PD-1 is the co-receptor for PD-ligands (PD-L1 and PD-L2) and, like CTLA-4, represses T-cell responses.21
FIGURE 59-7
The process of regulating T-cell (CD4+ and CD8+) activation involves engagement of the TCR/CD4 or CD8 with the foreign protein-HLA molecule and of several co-stimulators of the co-stimulatory pathway. The constitutively expressed CD28 co-receptor drives proliferation of naïve T-cells when engaged by its ligand B7-1. Inducible CTLA-4 (CD154) co-regulator acts to regulate this process negatively along with its APC-bound B7-2 ligand. Other CD28 family co-receptors include inducible ICOS that signals for proinflammatory IL-4 and IL-10 effector responses and PD-1 that has a negative regulatory effect on the T-cell. The APC is made “better” by a reciprocal activation between T-cells and APCs and the phenomena of “licensing” in which innate receptors (PAMP—Toll receptor) stimulate B7 expression. This has the effect also on the T-cell of inducing CD40 ligand (CD154). CD40 on the APC’s membrane drives additional B7 expression and elaboration of IL-12.

A second family of co-stimulatory molecules belong to the tumor necrosis factor (TNF) super family.22 The prototypic pathway is represented by the co-stimulatory receptor CD40 on APC binding to CD40 ligand (CD154) on T-cells. By reciprocal activation, CD154 on T-cells makes the APC “better” and is thought to “license” additional APCs to participate in the T-cell activation process. CD154 can also interact with CD40 expressed by CD8+ T-cells thereby providing direct help from CD4+ to CD8+ T-cells.23 Moreover, transplantation immunobiology is influenced by the innate immunity directors against substances from microbes, including bacteria, viruses, and fungi. These substances, often nucleic acids, are called pathogen-associated molecular patterns (PAMPs). PAMPs can bind to PAMP-receptors on APCs called TLRs. This engagement has the effect of strengthening the APC response, in part by increasing the expression of co-stimulatory molecules and proinflammatory cytokines, thereby strengthening signal 2.
Additional co-stimulatory molecules were recently discovered and alternative therapeutic approaches are being evaluated in experimental models.24,25 In addition to promoting full T-cell activation, co-stimulatory signals are also crucially involved in cellular interactions between T and B cells, in which B cells receive help from T-cells to become fully activated and differentiate in memory B cells and antibody-producing plasma cells.
Early cell-mediated rejection (CMR) begins with activation of CD8+ cells that bear TCR molecules which fit and engage donor-specific peptide-HLA Class I complexes on donor APCs. In the presence of help from CD4+ T-cells, they become cytolytic lymphocytes (CTLs) and directly attach to and kill graft endothelial and parenchymal cells bearing the identical donor peptide-HLA Class I complex presented to them by the APC “activators.” This is known as the direct effector pathway (distinct from direct presentation of HLA by donor APC) since the CD8 cell directly kills the allotarget. It is responsible for most acute CMR early (in the first few months) after transplant. In the presence of calcium, the protein perforin polymerizes onto the Class I bearing target cell and causes 16- to 20-nm pores to open in the cell membrane and allowing the CTL to release its granular content, including granzyme B and other cytotoxic molecules directly into the target cell, causing its apoptosis. Alternatively, apoptosis (programmed cell death) can also be stimulated in the target cell by interaction of the lymphocyte Fas ligand with the APO-1/Fas receptor of the target cell. Second messengers are elicited that activate endonucleases and proteases to cause fragmentation of DNA and thus organize the dissolution of the Class I bearing donor target cell (Fig. 59-8).
FIGURE 59-8
Complicated scheme of allograft rejection. CD8+ lymphocytes become activated by donor-derived dendritic cells transplanted with the allograft. These cells enter a direct alloresponse pathway by proliferating into CTLs. The CTLs recognize and attach to Class I HLA donor peptide molecules on the surface of allograft cells. They directly kill the allotarget through cell-mediated cytotoxicity by injection of proteolytic granules, creating perforin-induced membrane pores and induction of apoptosis. CD4+ T-cells are activated by host APCs that engulf donor protein shed from the allograft. The host APCs process the donor protein into surface-bound Class II HLA peptide molecules. The CD4 cells follow an indirect pathway in the alloresponse by proliferating and secreting growth factors plus proinflammatory cytokines. This indirect paracrine function augments CTL proliferation, attracts cellular mediators of inflammation-like macrophages and drives B cell central humoral immunity.

Unlike the CD8+ T-cells, the CD4+ T-cells primarily follow an indirect effector pathway (distinct from indirect presentation of HLA by recipient APC) by secreting various cytokines that drive inflammation and graft loss (Fig. 59-9). IL-2 increases the expression of its own receptors (IL-2R) on CD4+ T-cells, driving their proliferation and further differentiation. Activated CD4+ T-cells secrete additional lymphokines, including interferon-γ (IFNγ), which along with IL-2 stimulates CD8+ CTLs to bind to the allograft cells presenting donor MHC protein molecules. CD4+ T-cells are very heterogeneous. Four major categories are now recognized: Th1, Th2, Th17, and regulatory T-cells (Tregs). Th1 cells are the main mediators of CMR. They produce IFNγ and favor IL-12 production by macrophages. Th2 cells make IL-4, 5, and 13 and promote humoral responses. Th17 produces IL-17, a cytokine recently discovered and involved in inflammation. In conditions where the immune response is partially inhibited, Tregs develop with the ability to inhibit immune responses.
In severe rejection, B cells join the alloresponse when they receive help from CD4+ T-cells (Fig. 59-6). CD4+ T-cells are activated by APCs to express CD40L (CD154). Ligand B cells expressing CD40 and MHC Class II receptors engage helper CD4+ T-cells and proliferate. B cells participate in the rejection of allografts by efficiently providing APC function to helper CD4+ T-cells and by elaboration of antibodies that (1) recognize and destroy the donor endothelial targets; (2) participate in antibody-dependent cytotoxicity (ADCC), or (3) stimulate the proliferation and migration of activated endothelial cells.26 In the former, the endothelial antigen/antibody complexes activate the “classical” complement pathway, causing C3 and C5 to cleave, inciting inflammation (C5a) and formation of the membrane attack complex (MAC), which is comprised of C5b complexed with C6, 7, 8, and 9 (C5b-9). The MAC causes vascular cell death by inducing pores to form in the cell membrane. When endothelial cells are activated or killed by complement, this in turn results in microvascular thrombosis and inflammation. ADCC describes the process by which antidonor IgG antibodies coating donor cells bind to the Fcγ RIII receptor of NK lymphocytes (NK cell). Cells of the innate immune system such as NK cells are also present in allografts during rejection. These may react with alloantigens because they constitutively express inhibiting receptors specific for self-HLA Class I molecules or they may engage alloantibody-bound target (donor) cells through their Fcγ receptor. NK cells can not only directly kill target cells by injecting proteolytic enzymes into their cytoplasm, but also modulate immunity by producing proinflammatory cytokines like IFNγ (Fig. 59-8).
With improvement in immunosuppression that limits activation of naïve T-cells, donor-reactive memory cells have been recognized as a previously underappreciated risk to the allograft. This group of donor-reactive cells has an “effector memory” phenotype. This is defined by an immediate and strong recall that is less sensitive to co-stimulation blockade. Memory cells are thought to arise from heterologous immunity. This occurs when there is a resemblance between microbial antigens and donor HLA complex antigens. Some CD4+ and CD8+ cells exposed to Epstein Barr, Herpes Simplex, and cytomegalovirus (CMV) become primed to recognize allogenic HLA molecules. Memory T-cell numbers are proportionately increased following lymphoablative treatment with rabbit ATG or Alemtuzumab (anti-CD52 Ab). It is unclear whether these memory cells are more resistant to depletion or whether they represent a conversion from naïve T-cells during repopulation. Memory CD4+ cells provide help for the alloresponse. They provide growth and proinflammatory factors that affect CD8+ T-cells and B-cell antibody production. They can illicit the proinflammatory response from nodal-bearing tissue remote from the allograft. Memory CD4+ cells recruit CD8+ cells that infiltrate the allograft across the endothelium. The memory CD8+ cells proliferate and recruit macrophages, neutrophils, and additional activated T effector cells into the donor organ.27 To date, memory T-cells continue to evade efforts of therapeutic targeting. LFA-1 is one target that is under study for this purpose. Memory T cells are very heterogeneous and have multiple functions.
AMR may be defined as a form of acute graft rejection phenotypically characterized by evidence of tissue damage mediated by antigraft antibodies as effectors. The International Society for Heart and Lung Transplantation Working Group has codified specific criteria for cardiac AMR.28,29 They include clinical evidence of DSA within the recipient, endomyocardial biopsy immunopathologic evidence of complement (specifically C4d and C3d) staining, and endothelial cell and macrophage activation (the latter assessed by CD68 staining). Similar features of AMR have been identified in the lung, although less consensus exists (Figs 59-9 and 59-10).30
FIGURE 59-10
Specific C4d deposition on a lung transplant allograft in a recipient with circulating HLA antibody is continuous and subendothelial in capillaries and small arterioles (left). Specific C4d has been seen on 31% of transbronchial biopsies in recipients with HLA-Ab. (Ionescu D.N., Transplant Immunology 15(2005) 63-68) (middle). In contrast, nonspecific staining of the interstitium and elastic layer is depicted (right). (Used with permission from K.R. McCurry, M.D.)

Although B/plasma cell production of DSA is the essential pathophysiologic mechanism underlying AMR, T-cell help directs and/or influences AMR;31,32 consequently, AMR should not be thought of as T cell-independent. DSA may be “preformed,” that is, present prior to transplantation (see discussion of crossmatching and percent reactive antibody [PRA]), or develop de novo posttransplantation. It has been shown that DSA are identified temporally prior to pathological evidence of AMR33 and the development of anticardiac myosin immune responses.34
Presensitization to HLA Class I or Class II antibodies predisposes to AMR.35 Those recipients who develop presensitized antibodies, especially donor-specific, risk AMR.36 In addition to anti-HLA antibodies, non-HLA antibodies, including those against self-antigens such as cardiac myosin, vimentin, and endothelial cells have been less often associated with AMR.37-39 Cardiac AMR occurs early (weeks to months) after transplant and, if avoided early, rarely occurs later.40 Classically, histological diagnosis of cardiac AMR has included evidence of endothelial swelling and activation macrophages in the graft plus confirming immunofluorescence or immunoperoxidase staining for immunoglobulin (IgG or IgM). While absence of immunofluorescence seems reasonable to rule out AMR, capillary swelling (63%) and macrophage vascular adherence (30%)41 and complement (C3d and C4d) do not. Non-AMR causes of C4d staining include organ reperfusion injury, immunosuppression, treatment with monoclonal antibodies, and viral infections.42 The distribution (diffuse vs localized) and intensity of the stains has not found a standardization for grading.
The incidence of AMR after heart or lung transplantation is uncertain due to a lack of universal screening in asymptomatic recipients. Symptomatic pure AMR without a component of acute cellular rejection occurs in 10 to 15% of cardiac transplant recipients, but AMR features have been reported in up to 40% of patients with acute cellular rejection (mixed rejection).43 Clinical symptoms of cardiac AMR are those common in heart failure using echo. A >25% decrease in ejection fraction and an increase in left ventricular mass distinguished AMR from CMR.44 Reduced R-wave voltage-conduction abnormalities, including bundle branch block, are electrocardiographic associates.
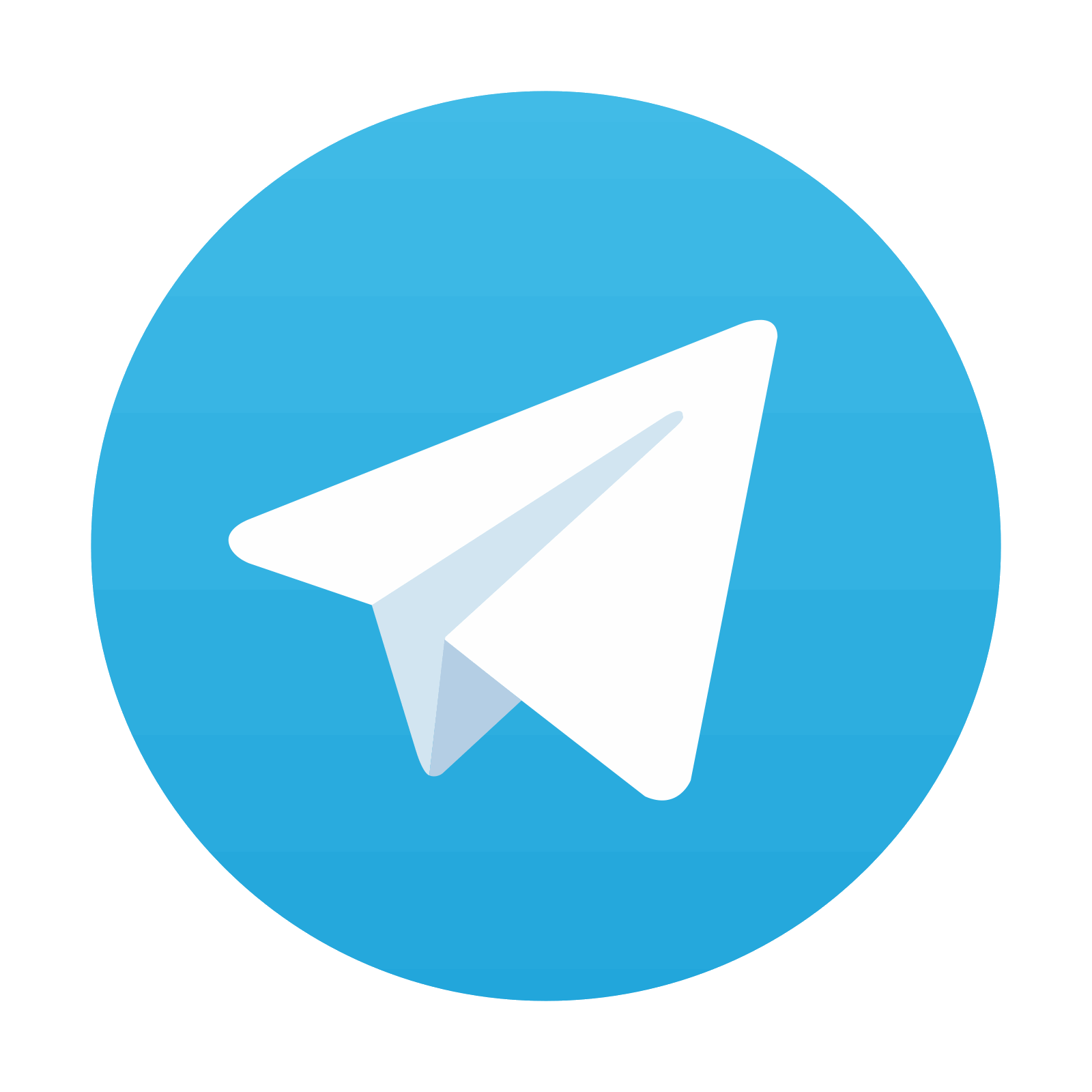
Stay updated, free articles. Join our Telegram channel
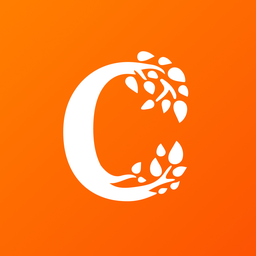
Full access? Get Clinical Tree
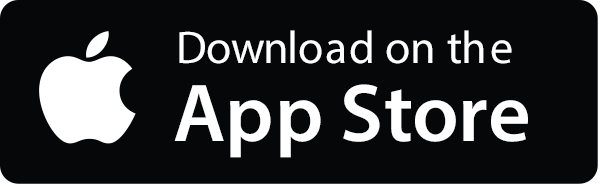
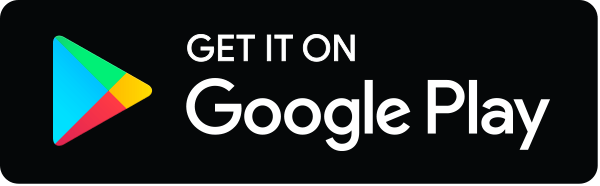