Chapter 7 Imaging Techniques
Plain Chest Radiography
Technical Considerations
The frontal (posteroanterior) (Figure 7-1) and lateral (Figure 7-2) projections are sufficient for most purposes in chest radiography. Other radiographic views are less frequently required, but they should not be overlooked because they may solve a particular problem quickly and cheaply. The lateral decubitus view is not, as its name implies, a lateral view. It is a frontal view taken with use of a horizontal beam and the patient in a side-lying position. Its main purpose is to demonstrate the movement of fluid in the pleural space (Figure 7-3). An adaptation of this view is the “lateral shoot-through” sometimes used in bed-bound patients: A lateral radiograph of the supine patient is taken to show an anterior pneumothorax behind the sternum (not always visible on a frontal chest radiograph) (Figure 7-4). If a pleural effusion is not loculated, it gravitates, to some extent, to the dependent part of the pleural cavity. Thus, in a decubitus patient, the fluid will layer between the chest wall and the lung edge. This view also may be useful for demonstrating a small pneumothorax, because the visceral pleural edge of the lung falls away from the chest walls in the nondependent hemithorax.
For the lordotic view, now rarely performed, the x-ray beam is angled 15 degrees cranially, either by positioning the patient upright and directing the beam up or by leaving the beam horizontal and leaning the patient backward. On this view, the lung apices are demonstrated free from the superimposed clavicle and first rib. It may be useful to differentiate pulmonary shadows from incidental calcification of the costochondral junctions (Figure 7-5).
Computed Tomography
An impressive and rapid improvement in CT hardware capability has occurred over the past decade. Most particularly, the advent of multiple-channel CT scanners has resulted in the ability to acquire simultaneous helical datasets. An accompanying increase in gantry rotation speed coupled with the reduction in the size of the individual detectors has resulted in the ability to acquire extremely detailed images in very short scan times. On the current “top specification” scanners from the major manufacturers, up to 320 channels are available, each with a detector size of as small as 0.5 mm. The entire thorax can now be scanned at submillimeter resolution in 1 to 2 seconds. Thus, spiral (also known as volume or helical) scanning entails continuous scanning and table movement into the CT gantry (Figure 7-6). The information is reconstructed into axial sections, perpendicular to the long axis of the patient, identical to conventional CT sections.
The analysis of what is frequently hundreds of individual images that are produced as the result of a single CT examination is undertaken on dedicated CT or PACS workstations. Postprocessing of these thin sections also allows the production of multiplanar reformats (MPRs), maximum and minimum intensity projections (MIPs and MinIPs), and angiographic images. Skeletal structures can be automatically removed, or surface-rendered images that mimic appearances familiar to the bronchoscopist can be produced with a few mouse clicks. These images are visually pleasing and allow an exquisite appreciation of anatomy. They also have a role in the planning of interventional procedures, including transbronchial needle biopsy and endoluminal stent insertion (Figure 7-7).
Section Thickness
Although a CT section is viewed as a two-dimensional image, it has a third dimension of depth. The depth, or section thickness, is determined by a combination of factors, depending on the exact parameters utilized, including focal spot size, thickness of the individual detector elements, and width of the x-ray beam collimation. Because a section has a predetermined thickness, each pixel has a volume and this three-dimensional element is referred to as a voxel. The computer calculates the average radiographic density of tissue within each voxel, and the final CT image consists of a representation of the numerous voxels (not individually visible without magnification) in the section. The single attenuation value of a voxel represents the average of the attenuation values of all of the various structures within the voxel. The thicker the section, the greater the chance that different structures will be included within the voxel and the greater the signal averaging that occurs. This is known as the partial volume effect; the easiest way to reduce this effect is to use thinner sections (Figure 7-8).
Window Settings
The window width determines the number of Hounsfield units to be displayed. Any densities greater than the upper limit of the window width are displayed as white, and any below the limit of the window are displayed as black. Between these two limits, the densities are displayed in shades of gray. The median density of the window chosen is the window center or level; this center can be moved higher or lower as desired, thus moving the window up or down through the range. The narrower the window width, the greater the contrast discrimination within the window. No single window setting can depict the wide range of densities encountered in the chest on a single image. For this reason, at least two sets of images are required to demonstrate the lung parenchyma and soft tissues of the mediastinum, respectively (Figure 7-9). Standard window widths and centers for thoracic CT vary between departments, but generally for the soft tissues of the mediastinum, a window width of 400 to 600 H and a center of +30 H is appropriate. For the lungs, a wide window of 1500 H and a center of approximately −500 H are usually satisfactory. For bones, the widest possible window setting at a center of +30 H is best.
High-Resolution Computed Tomography
Technical Considerations
Narrow collimation of the x-ray beam reduces volume averaging within the section and so increases spatial resolution compared with standard 10-mm collimation. For routine HRCT scanning, 1.50-mm beam collimation generally is regarded as optimal. Narrow collimation has a marked effect on the appearance of the lungs, notably the vessels and bronchi—the branching vascular pattern seen particularly in the midzones on standard 10-mm sections has a more nodular appearance with narrow sections, because shorter segments of the obliquely running vessels are included in the section. In addition, parenchymal details become more clearly visualized (Figure 7-10).
In HRCT lung imaging, a high-spatial-frequency algorithm is used to take advantage of the inherently high-contrast environment of the lung. The high-spatial-frequency algorithm (also known as the edge-enhancing, sharp, or formerly “bone” algorithm) reduces image smoothing and makes structures visibly sharper but at the same time makes image noise more obvious (see Figure 7-10).
The size of the patient has a direct effect on the quality of the lung image—the larger the patient, the more conspicuous the noise, which is seen as granular streaks because of increased x-ray absorption by the patient. This artifact is particularly evident in the posterior lung adjacent to the vertebral column. The phenomenon of aliasing results in a fine, streaklike pattern radiating from sharp, high-contrast interfaces. The severity of the aliasing artifact is related to the geometry of the CT scanner, and, unlike quantum mottle, aliasing is independent of the radiation dose. These artifacts are exaggerated by the nonsmoothing, high spatial-resolution reconstruction algorithm but do not mimic normal anatomic structures and are rarely severe enough to obscure important detail in the lung parenchyma (Figure 7-11).
A limited number of scans taken at end expiration can reveal evidence of air trapping caused by small airway disease, which may not be detectable on routine inspiratory scans. Areas of air trapping range from a single secondary pulmonary lobule to a cluster of lobules that give a patchwork appearance of low attenuation areas adjacent to higher attenuation, normal lung parenchyma (Figure 7-12).
Clinical Applications of High-Resolution Computed Tomography
In patients with clinical and lung function evidence of diffuse lung disease, HRCT is now central in the diagnostic workup, with clinical performance greatly exceeding that of plain chest radiography and may obviate the need for lung biopsy. In the original study that compared the diagnostic accuracy of chest radiography and CT in the prediction of specific histologic diagnosis in patients with diffuse lung disease, Mathieson and associates showed that three observers could make a confident diagnosis in 23% of cases on the basis of chest radiographs and in 49% of cases with use of CT; the correct diagnosis was made in 77% and 93% of these readings, respectively (Figure 7-13).
A number of subsequent early HRCT studies acted as the forerunners of a large body of work that has established HRCT as a cornerstone in the assessment of patients suspected of having diffuse lung disease but for whom the clinical features and appearance on the chest radiograph do not allow a confident diagnosis to be made. A number of diffuse lung diseases can have a “diagnostic” appearance on HRCT when findings are interpreted by experienced chest radiologists; such diseases include fibrosing alveolitis, sarcoidosis, Langerhans cell histiocytosis, lymphangioleiomyomatosis, pneumoconiosis, and hypersensitivity pneumonitis (Figure 7-14). An intriguing observation is that the ability of HRCT to allow observers to provide correct histospecific diagnoses seems to be maintained in advanced end-stage disease.
Much interest has been shown in defining the role of HRCT in staging disease activity, particularly for fibrosing alveolitis, in which cellular histology indicates disease activity and is used to predict both responses to treatment and prognosis. As shown by more recent evidence, a predominance of ground glass opacification in fibrosing alveolitis predicts a good response to treatment and increased actuarial survival compared with patients with a more reticular pattern, which denotes established fibrosis. Similar observations about the potential reversibility of disease can be made with use of HRCT in patients who have sarcoidosis, in whom a ground glass or a nodular pattern predominates. In other conditions, the identification of ground glass opacification on HRCT, although nonspecific, almost invariably indicates a potentially reversible disease—for example, extrinsic allergic alveolitis, diffuse pulmonary hemorrhage, and Pneumocystis jiroveci pneumonia (Box 7-1). An important exception is bronchoalveolar cell carcinoma, in which areas of ground glass opacification that merge into areas of frank consolidation or a more nodular pattern may be seen. Another caveat applies with the situation in which fine, intralobular fibrosis is seen on HRCT as widespread ground glass opacification; in this rare occurrence, evidence of traction bronchiectasis usually is present within the areas of ground-glass opacification.
Box 7-1
Causes of Ground Glass Opacification
In patients for whom lung biopsy is deemed necessary, HRCT may be invaluable to indicate which type of biopsy procedure is likely to be successful in obtaining diagnostic material. The broad distinction between peripheral disease versus central and bronchocentric disease is easily made on HRCT. Thus, disease with a subpleural distribution, such as fibrosing alveolitis, is most unlikely to be sampled by transbronchial biopsy, whereas diseases with a bronchocentric distribution on HRCT, such as sarcoidosis and lymphangitis carcinomatosa, are consistently accessible to transbronchial biopsy. In patients for whom an open or thoracoscopic lung biopsy is contemplated, HRCT assists in determining the optimal biopsy site. Pathologic examination of a lung biopsy specimen can still justifiably be regarded as the final arbiter of the presence or absence of subtle interstitial lung disease. Because HRCT images provide a kind of “in vivo big picture,” many lung pathologists now combine the imaging and pathologic information before assigning a final diagnosis, and in many centers, the benefits of a team approach to the diagnosis of diffuse lung disease are recognized. The indications for HRCT that have been developed over the past 20 years are summarized in Box 7-2.
Box 7-2
Indications for High-Resolution Computed Tomography of the Lungs
To narrow the differential diagnosis or to make a histospecific diagnosis in patients with obvious but nonspecific radiographic abnormalities
To detect diffuse lung disease in patients with normal or equivocal radiographic findings
To elucidate unexpected pulmonary function test results
To investigate the underlying problem in patients presenting with hemoptysis
To evaluate disease reversibility, particularly in patients who have fibrosing alveolitis
Magnetic Resonance Imaging
Mediastinal and Chest Wall Imaging
The most common indications for the use of MRI in respiratory disease are for investigation of neoplastic disease, most commonly bronchogenic carcinoma. In addition to the primary disease, secondary complications such as cerebral secondaries, spinal metastases, and retroperitoneal fibrosis all lend themselves to evaluation by MRI. MRI also permits assessment for invasion of mediastinal structures such as the major airways, heart and great vessels, chest wall, and diaphragm and allows differentiation among different forms of soft tissue, fluid, hemorrhage, local hematoma formation, and aneurysms (Figures 7-15 and 7-16). With modern multichannel CT techniques, MRI now holds relatively little advantage over CT in assessing chest wall invasion, except with superior sulcus tumors. However, MRI does provide superb anatomic detail without subjecting the patient to radiation exposure—an important consideration in the pediatric age group, in which a number of follow-up studies may be required (Figure 7-17). The disadvantage of MRI in the very young child is the necessity for general anesthesia in many cases.
Magnetic Resonance Angiography
Magnetic resonance also can be used to demonstrate vascular anatomy by differential visualization of flowing blood and stationary tissue; this may be achieved with or without intravenous MRI contrast agents. Generally, the use of contrast increases the signal returned from blood, increases the signal-to-noise ratio, and allows acquisition times to be shorter. This modality, known as magnetic resonance angiography (MRA), can be used to look at venous or arterial flow, together or separately (see Figure 7-17).
Vascular Malformations and Congenital Anomalies
Increasing evidence suggests that MRI can clearly define a number of vascular and developmental anomalies of the lungs by combining anatomic and flow studies. Such anomalies include the scimitar syndrome, hypogenetic lung syndrome, pulmonary artery agenesis, bronchopulmonary sequestration, and vascular malformations (see Figure 7-17).
Pulmonary Angiography
The technique of pulmonary angiography involves fluoroscopically directed insertion of a guidewire followed by a modified pigtail catheter into the right and then the left main pulmonary arteries in turn, with injection of a nonionic contrast administered at an appropriate flow rate. At least two views per side are required, with additional oblique or magnification views as necessary. Catheter access usually is through the femoral vein, with use of the internal jugular and subclavian veins as possible alternatives. Most departments undertake angiography with digital subtraction vascular equipment (Figure 7-18). Problems with misregistration artifact, inherent in digital subtraction systems and caused by respiratory or cardiac cycle phase differences between the mask image and the contrast image, usually can be overcome. Crossing the tricuspid valve may induce an arrhythmia that usually is transient. Therefore, electrocardiogram (ECG) monitoring is mandatory, and the use of prophylactic antiarrhythmic agents or temporary pacing-wire insertion is common practice in some centers. Right-sided heart catheter pressure measurements and gas analysis also may be undertaken.
Bronchial Artery Embolization
Fiberoptic bronchoscopy often is advocated before bronchial artery embolization to establish the site of hemorrhage. However, a large-volume hemoptysis almost invariably results in vigorous coughing, thereby spreading blood throughout the bronchial tree, which makes localization impossible. CT angiography also is a useful preliminary investigation, delineating bronchial artery anatomy, guiding intervention, and sometimes localizing the lobe or segment from which the bleeding originates. Few criteria exist to determine which angiographically demonstrated bronchial arteries should be embolized. Guidelines are particularly relevant when several bronchial arteries have been identified and the site of hemorrhage is not obvious from previous thoracic imaging. Embolization is directed at the vessels considered most likely to be the source of hemorrhage (Figure 7-19). Bronchial arteries of diameter greater than 3 mm may be considered to be pathologically enlarged. In patients with diffuse, suppurative lung disease, most commonly cystic fibrosis, attempts are made to embolize all significantly enlarged bronchial arteries bilaterally. If no abnormal bronchial arteries are identified, a systematic search is made for aberrant bronchial arteries. When a patient continues to experience hemoptysis after embolization, all suspicious systemic arteries should be examined for a source of bleeding, and it may be necessary to angiographically investigate the pulmonary circulation for a source of bleeding.
Superior Vena Cava Stenting
The percutaneous placement of metallic stents for the treatment of SVCO has several attractions. With increasing experience, reliable and successful palliation of SVCO has been reported with use of various stent designs. A superior venacavagram is necessary to identify the length of the stenosis and its site in relation to the confluence of the brachiocephalic veins and the right atrium. Identification of intraluminal thrombus or tumor may require thrombolysis before stent insertion, or the use of a covered stent. After balloon dilatation of the superior vena cava stricture, the stent is positioned across the stricture, and a postplacement venacavagram is performed to confirm free flow of blood into the right atrium (Figure 7-20). Subsequent to angioplasty and stent placement, relief of SVCO symptoms usually is rapid and dramatic. Recurrence of symptoms may be caused by venous thrombosis or tumor progression. Although rupture of the superior vena cava at the time of angioplasty is a risk, this complication seems to be extremely rare, possibly because of the tamponade provided by surrounding tumor or postirradiation fibrosis.
Normal Radiographic Anatomy
Mediastinum and Hilar Structures
The mediastinum is conventionally divided into superior, anterior, middle, and posterior compartments (Figure 7-21). The practical benefit of use of these arbitrary divisions is that specific mediastinal pathologies show a definite predilection for individual compartments (e.g., a superior mediastinal mass most frequently is caused by intrathoracic extension of the thyroid gland; a middle mediastinal mass usually results from enlarged lymph nodes). However, localization of a mass within one of these compartments does not normally allow a specific diagnosis to be made, and neither do the arbitrary boundaries preclude disease from involving more than one compartment.
Only the outline of the mediastinum and the air-containing trachea and bronchi (and sometimes esophagus) is clearly seen on a normal posteroanterior chest radiograph. On a chest radiograph, the right brachiocephalic vein and superior vena cava form the right superior mediastinal border. This border usually is vertical and straight (in contrast with the situation in which right paratracheal lymphadenopathy is present, when the right superior mediastinal border tends to be undulate), and it becomes less distinct as it reaches the thoracic inlet. The right side of the superior mediastinum can appear to be considerably widened in patients who have an abundance of mediastinal fat (Figure 7-22); such persons often have prominent cardiophrenic fat pads. The mediastinal border to the left of the trachea above the aortic arch is the result of summation of the left carotid and left subclavian arteries, together with the left brachiocephalic and jugular veins. The left cardiac border consists of the left atrial appendage, which merges inferiorly with the left ventricle. The silhouette of the heart should always be sharply outlined. Any blurring of the border results from loss of immediately adjacent aerated lung, usually by collapse or consolidation.
The trachea and main bronchi should be visible through the upper and middle mediastinum. The trachea is rarely straight and often is to the right of the midline at its midpoint. In older persons, the trachea may be markedly displaced by a dilated aortic arch below. In approximately 60% of normal subjects, the right wall of the trachea (the right paratracheal stripe) can be identified as a line of uniform thickness (less than 4 mm in width); when visible, it excludes the presence of any adjacent space-occupying lesion, most usually lymphadenopathy. The angle between the left and right main bronchi, which forms the carina, usually is somewhat less than 80 degrees. Splaying of the carina is a relatively crude sign of subcarinal disease, in the form of either a massive subcarinal lymphadenopathy or a markedly enlarged left atrium. A more sensitive sign of subcarinal disease is obscuration of the upper part of the azygoesophageal line, which usually is visible in its entirety on a chest radiograph with good penetration (Figure 7-23). The origins of the lobar bronchi, when they are projected over the mediastinal shadow, usually can be identified, but segmental bronchi within the lungs generally are not seen on plain radiography.
Pulmonary Fissures, Vessels, and Bronchi
The two lungs are separated by the four layers of pleura behind and in front of the mediastinum. The resultant posterior and anterior junction lines often are visible on frontal chest radiographs as nearly vertical stripes, the posterior junction line lying higher than the anterior (Figure 7-24). Because these junction lines are not invariably seen (their visibility is largely dependent on whether the pleural reflections are tangential to the x-ray beam), their presence or absence is not usually of significance.
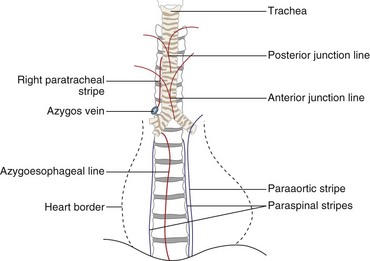
Figure 7-24 Diagram showing some of the mediastinal lines and stripes frequently seen on a frontal chest radiograph.
Diaphragm and Thoracic Cage
Apparent pleural thickening along the lateral chest wall in the middle zones is a frequent observation in obese patients; it is caused by subpleural fat bulging inward. Deformities of the thoracic cage may cause distortion of the normal mediastinum and so simulate disease. One of the most common deformities is pectus excavatum, which, by compressing the heart between the depressed sternum and vertebral column, causes displacement of the apparently enlarged heart to the left and blurring of the right heart border (Figure 7-25). A similar appearance may arise with an unusually straight thoracic spine, referred to as straight back syndrome.
Anatomy of the Lateral Chest Radiograph
Consistent viewing of lateral chest radiographs in the same orientation, whether a right or a left lateral projection, improves the ability to detect deviations from normal. In the lateral view, the trachea is angled slightly posteriorly as it runs toward the carina, and its posterior wall is always visible as a fine stripe (Figure 7-26
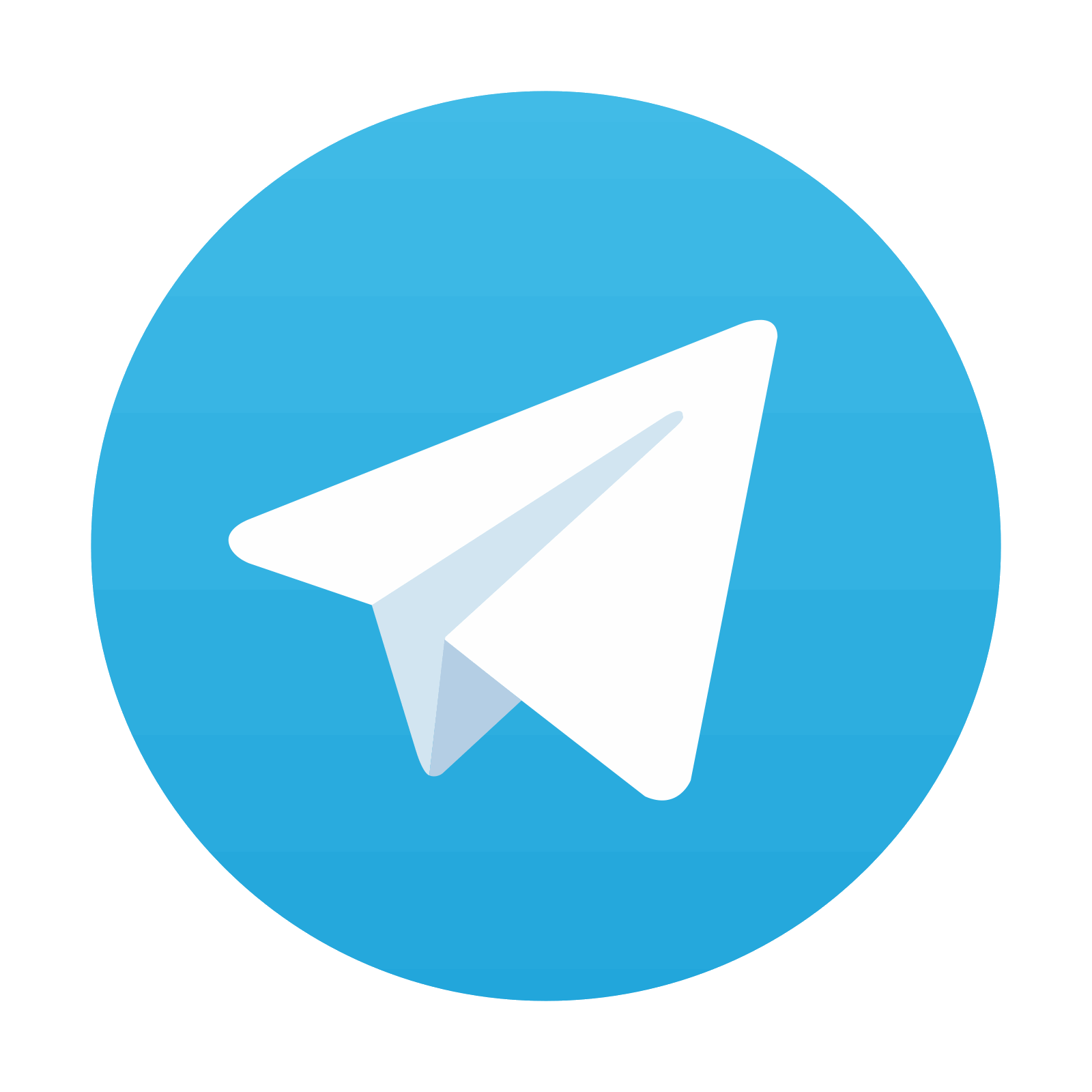
Stay updated, free articles. Join our Telegram channel
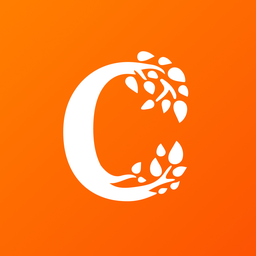
Full access? Get Clinical Tree
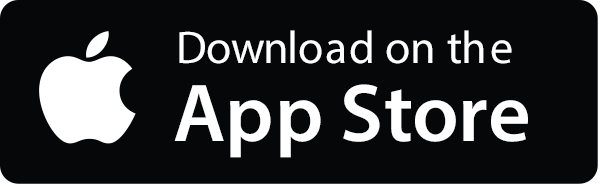
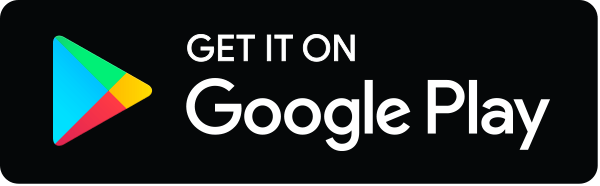