Echocardiography has undergone major progress in recent years. While standard cross sectional echocardiography is still the most often used routine method for screening the anatomy of congenitally malformed hearts, and for evaluation of cardiac function, the use of echocardiography has extended to the operating theatre to review surgical results, to monitor interventions, and to evaluate ventricular function in a much more sophisticated way than conventional echocardiography. These recent developments include intracardiac echocardiography, intra-operative echocardiography using either transoesophageal or intracardiac transducers, real-time three-dimensional echocardiography, myocardial or tissue Doppler, and two-dimensional strain. In this part of our chapter devoted to imaging, I focus on myocardial Doppler and two-dimensional strain based on speckle tracing. These two techniques offer new methods for functional assessment of the heart, and are especially useful in evaluating regional and global function of both the right and left ventricle, as they allow for assessment of ventricular function irrespective of the ventricular shape.
BASIC PRINCIPLES
For myocardial Doppler, the same physical principles apply as in blood pool Doppler. The velocities of the flowing blood are high, but have low amplitude. These velocities are in the region of 1 m/sec across normal heart valves, and may increase to 6 m/sec across stenosed cardiac valves or narrowed great vessels. When blood pool sonographic Doppler was developed, special filters were used to suppress the signal having low velocity and high amplitude originating from movement of the myocardium. These myocardial velocities typically are in the range of around 10 cm/sec at rest, and may increase to 30 cm/sec under catecholamine-induced stress. It was demonstrated in 1989 1 that the myocardial velocities could be measured by pulsed Doppler sonography, and offered clinically useful information. Filters were developed, therefore, which suppress the high velocities at low amplitude originating from the flow of blood ( Fig. 18C-1 ). After it had become evident that assessment of myocardial velocities was a useful clinical tool, colour Doppler imaging of the myocardium was also developed. 2 The velocity curves obtained from colour Doppler imaging are smoother than those from pulsed Doppler, and can be easily measured ( Fig. 18C-2 ). Myocardial velocities, nonetheless, may be influenced by factors other than myocardial contraction alone, such as the overall motion of the heart during breathing, cardiac rotation, and motion induced by tethering to adjacent segments. 3 Some of these problems of measuring myocardial velocities can be overcome by strain and strain rate imaging, which is calculated as the gradient in myocardial velocities over a given distance, usually 1 cm.


Strain, notated as ε, is dimensionless, as it is calculated as a quotient of velocities ( Fig. 18C-3 ). The first derivative of strain is strain rate. Strain provides information about the overall deformation of the myocardium, while strain rate describes how this deformation occurs over time. Both give different information, first on the degree of deformation, and second, on the time course of this deformation.

The major limitation of any Doppler measurement of myocardial velocities and its derivatives, such as strain and strain rate, is the dependency of the Doppler technique on the angulation of the insonating beam. This is a particular problem in patients with congenitally malformed hearts with abnormal chamber topography and dilated or hypertrophied ventricles, which make it very difficult to place the echocardiographic transducer correctly to align the beam with the myocardial wall.
A novel alternative method to Doppler-based measurement of strain is speckle tracking, which allows estimation of the velocity vector, rather than the velocity component, along the line of the image. This method is based on tracking patterns of radio-frequency imaging. 4 The algorithm finds the velocity vector by tracking patterns in the radio-frequency image between consecutive frames during the cardiac cycle, and thus makes it possible to find the in-plane frame-to-frame position of a pixel relative to its initial position ( Fig. 18C-4 ). This technique requires high-quality b-mode images recorded at frame rates of at least 60 frames per second. 5 With this method, deformation can be assessed in two directions simultaneously, such as radial and longitudinal. By convention, this method has been named two-dimensional strain.

EXPERIMENTAL AND CLINICAL VALIDATION OF MYOCARDIAL DOPPLER AND SPECKLE TRACKING
Measurement of myocardial velocities, assessment of longitudinal strain by estimating myocardial velocity gradients, and two-dimensional strain by speckle tracking, have all been validated experimentally. 6–8 These experimental validations were achieved by comparison of the novel echocardiographic techniques to pressure-volume loops obtained using conductance catheterisation, or using sonomicrometric crystals on the epicardium to quantify myocardial deformation.
Gorcsan et al were the first to validate colour-coded myocardial velocities for the assessment of regional and global left ventricular function. 6 Peak systolic myocardial velocities increased with dobutamine and decreased with esmolol, while simultaneously measured end-systolic elastance, by use of a conductance catheter, correlated well with these changes. Regional left ventricular peak systolic velocities mirrored changes in myocardial length as measured by the crystals placed in the respective myocardial segments. The first clinical validation of myocardial Doppler was achieved by comparing transmural myocardial biopsies obtained during coronary arterial bypass surgery with myocardial Doppler tracings. 9 The histological findings were compared to systolic and diastolic myocardial velocities measured the day before surgery, revealing significant relationships between systolic and early diastolic myocardial velocities and beta-adrenergic receptor density, and the proportion of interstitial fibrosis. The study proved that systolic and diastolic myocardial velocities in humans are dependent on the number of functioning myocytes, and the density of myocardial β-adrenergic receptors.
Doppler-derived longitudinal strain was validated experimentally using ultrasonic crystals placed near the left ventricular apex and base. 7 During ischaemia induced by occlusion of the anterior interventricular artery, the apical myocardium became dyskinetic. Doppler-derived longitudinal strain reacted the same way as the crystals. Thus, measurement of myocardial deformation by strain was shown to detect changes in regional motion affected by ischaemia. The study also demonstrated the load-dependency of myocardial velocities and Doppler-derived strain, and confirmed the techniques to be dependent on the angle of insonation, thus limiting their clinical applications.
Because of this, speckle tracking was developed and validated to assess deformation independent of the angle of insonation. 8 Again, sonomicrometric crystals were used as reference, and their changes during inotropic modulation and ischaemia were compared to changes in radial and longitudinal strain. Good agreements were obtained for measurements of both radial and longitudinal strain, and good correlations shown between ultrasonic strain as revealed by speckle tracking and deformation of the crystals.
Although these studies demonstrated the accuracy of myocardial velocities derived by Doppler and speckle tracking, as well as strain, the methods were found to be load-dependent. As such, they offered little advantage over traditional indexes, such as ejection or shortening fraction for evaluating global ventricular function. 10 Measurements of myocardial contraction during the isovolumic period are likely to be more robust. One of the commonest measured isovolumic indexes remains dP/dt max, which can only be assessed by invasive techniques. 11 After we had noticed that the ventricular myocardial velocity during isovolumic contraction increased more markedly than the peak systolic velocity during dobutamine stress, we used myocardial Doppler to measure the acceleration of the myocardium during isovolumic contraction, in other words isovolumic acceleration. Subsequently, we validated experimentally isovolumic acceleration as an index of contractile function, comparing it to myocardial acceleration and velocities measured during the ejection phase. 12 As the independent index of contractility for comparison of myocardial Doppler data, we used pressure-volume analysis, derived by conductance catheter, measuring in this way end-systolic and maximal elastance in both ventricles. 13,14 Our studies confirmed that small changes in contractile function during beta blockade or stimulation of beta receptors could be detected by measuring isovolumic acceleration ( Fig. 18C-5 to18C-7 ). Importantly, changes in pre- and after-load in a physiological range ( Figs. 18C-8 and 18C-9 ) did not affect isovolumic acceleration, while the myocardial Doppler-derived indexes of the ejection phase were all influenced significantly by these changes. These experimental findings of the independence of measurements of isovolumic acceleration from conditions of loading were subsequently confirmed in healthy humans, as well as in patients with abnormal loading conditions. 15,16





We also performed a clinical validation of isovolumic acceleration in patients with transposition subsequent atrial redirection procedures, in which the morphologically right ventricle continues to support the systemic circulation. 17 As some of these patients develop systemic ventricular dysfunction in the second, third, or fourth decade oflife, it is of clinical interest to assess prospectively their contractile reserve. From 80 such adults, we recruited 12 who simultaneously underwent a study using conductance catheters and myocardial Doppler. At rest, as well as during β-receptor stimulation using dobutamine, we analysed pressure-volume relations and isovolumic acceleration. Both parameters of contractile function increased significantly during β stimulation ( Fig. 18C-10 ). This showed, first, that isovolumic acceleration can detect changes in contractile function in the human, and second, that measurement of the contractile reserve in patients with a systemic morphologically right ventricle has a clinical value. Critics have claimed that isovolumic acceleration may not be due to a ventricular event. We have demonstrated experimentally, using simultaneous recordings of right ventricular pressure and myocardial Doppler, that isovolumic acceleration occurs immediately before the ventricle builds up pressure to eject blood, which may be an explanation for the relative insensitivity of isovolumic acceleration to changes in conditions of ventricular loading ( Fig. 18C-11 ). In a patient with complete heart block, we found no signal of isovolumic acceleration during atrial contraction when we had turned off the pacemaker. Isovolumic acceleration reappeared with the first ventricular escape beat ( Fig. 18C-12 ).



Experimental and clinical data suggests, therefore, that isovolumic acceleration provides a robust measurement of left or right ventricular contractile function, with a sensitivity approaching or exceeding those indexes traditionally measured using invasive techniques. During these experiments, we also noticed a dependency of isovolumic acceleration on the heart rate, which increased during atrial pacing. This suggested that we could non-invasively assess the force-frequency relation. 18 This force-frequency relation is a fundamental physiological parameter of myocardial function. 19 Figure 18C-13 illustrates a typical example of this ventricular phenomenon. We found that isovolumic acceleration increased significantly with heart rate during incremental pacing in both ventricles, mirroring changes in dP/dt max . The normal pattern is for increasing development of force during incremental tachycardia to a point of maximal development, beyond which force then decreases with ongoing increases in heart rate. 19,20 An abnormal force-frequency relationship has been found in patients with dilated and hypertrophic cardiomyopathies, and in those with cardiac failure. 21,22 Until now, the force-frequency relation has either been assessed on the explanted myocyte, or invasively using dP/dt max as a measurement of contractile force. 23,24 Subsequently the force-frequency relation could be measured non-invasively by assessing isovolumic acceleration in normal subjects, as well as patients with functionally univentricular hearts. 25

NORMAL CARDIAC FUNCTION ASSESSED BY MYOCARDIAL DOPPLER AND TWO-DIMENSIONAL STRAIN
The myocardial Doppler-derived isovolumic acceleration, systolic velocities, and strain, as well as two-dimensional strain, change with age. 26 Thus, appropriate reference values need to be used for those of different ages. 27,28 By convention, systolic velocities, caused by shortening of the myocytes, are displayed as positive waves above the zero line, and diastolic velocities, due to relaxation of the myocytes, are displayed as negative waves below the zero line ( Fig. 18C-14 ). For longitudinal strain, commonly assessed in the four-chamber view, the convention is different. Deformation during systolic shortening of the myocytes is displayed as a negative curve ( Fig. 18C-15 ) below the zero line, while deformation during diastolic lengthening is displayed as a positive curve. This is because most cardiologists imaging adults look at the heart upside down in the four-chamber view. In contrast, most cardiologists imaging congenitally malformed hearts use anatomic views in the four-chamber view. Despite this difference, the convention of displaying velocities of shortening above the zero line as positive velocities, and velocities of lengthening of myocardial fibres as negative, has been accepted by cardiologists evaluating congenitally malformed hearts.


Myocardial velocities of shortening can be displayed in a different way, which facilitates recognition of abnormalities of ventricular mural motion. The integral of myocardial velocity data displays the distance a piece of myocardium covers during movement from base to apex. Online calculation makes possible instant recognition of abnormalities of wall motion ( Fig. 18C-16 ). The different distances are colour-coded. As only the velocities directed from base to apex are integrated, any piece of the myocardium which does not shorten from base to apex in systole remains grey. Thus, areas of abnormal motion, which paradoxically lengthen in systole when the remainder of the myocardium is contracting, can be readily identified ( Fig. 18C-17 ).


In a normal pulsed wave Doppler, or colour Doppler, myocardial velocity tracing, it is possible to discern a positive velocity during isovolumic contraction, a positive systolic velocity, known as the s-wave, an early negative diastolic velocity, the e-wave, and a negative diastolic velocity during atrial contraction, the a-wave. In a normal morphologically right ventricle, there is no discernible isovolumic relaxation time, while a small notch can be seen in the tracing of myocardial velocity obtained from the left ventricle, the notch identifying the end of isovolumic relaxation, which begins immediately following the crossing of the zero line by the velocity of the s-wave ( Figs. 18C-18 and 18C-19 ).


The normal values for isovolumic acceleration, myocardial velocities, and strain and strain rate are listed in Table 18C-1 . In the normal heart, there is a gradual decrease of systolic and diastolic myocardial velocities from base to apex ( Fig. 18C-20 ). In contrast, longitudinal strain, which represents a velocity gradient, changes little from base to apex in either ventricle ( Fig. 18C-21 ).
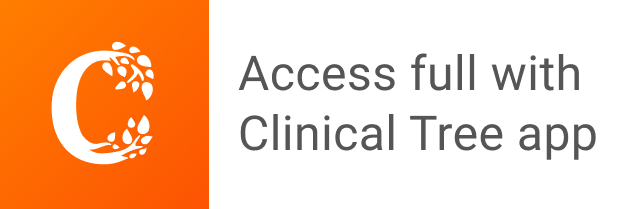