Hypoxic Respiratory Failure
GENERAL PRINCIPLES
Definition
Respiratory failure describes a set of conditions impairing delivery of oxygen to the tissues or removal of carbon dioxide from the tissues.
Classification
• Respiratory failure can be classified under many different schemas, each having its advantages.
• Classification by timing of onset, underlying etiology and/or anatomic area is useful when determining a differential diagnosis.
• Understanding the pathophysiology of respiratory failure and applying physiologic principles can guide the physician in developing a differential diagnosis and administering timely treatment to the patient.
• On the basis of pathophysiologic abnormalities, there are four types of respiratory failure.
Type 1: hypoxic respiratory failure.
Type 2: hypercapnic respiratory failure (see Chapters 6, 10, and 24).
Type 3: postoperative or atelectatic respiratory failure.
Type 4: circulatory shock-associated respiratory failure associated with hypoperfusion of respiratory muscles.
Etiology
• Causes of hypoxemic respiratory failure are shown in Figure 5-1.1
• The primary derangement in acute hypoxic respiratory failure is an inability of the cardiopulmonary system to deliver adequate oxygen supply to the tissues.
• Clinically, this can be further defined as the partial pressure of arterial oxygen (PaO2) <60 mm Hg.
• It is also important to differentiate hypoxia from hypoxemia.
• Hypoxia occurs when tissues are not adequately supplied with sufficient oxygen to accomplish cellular respiration.
• Hypoxemia is characterized by a decrease in the content of oxygen in arterial blood. This includes both oxygen bound to hemoglobin and oxygen dissolved in the blood.
• Hypoxemia is, therefore, a form of hypoxia.
• There are four basic classes of hypoxia:
Hypoxemic hypoxia: low arterial oxygen content with impaired oxygen delivery.
Anemic hypoxia: low circulating hemoglobin concentration with impaired oxygen delivery.
Circulatory hypoxia: low cardiac output with impaired oxygen delivery.
Cytotoxic hypoxia: poisoning with cyanide where oxygen is delivered to the tissues but cannot be used.
FIGURE 5-1. Etiology and approach to hypoxemic respiratory failure. (From Kollef M, Isakow W. The Washington Manual of Critical Care. 2nd ed. Philadelphia, PA: Lippincott Williams & Wilkins; 2012:42.)
Pathophysiology
• Hypoxemia results from one of six basic pathophysiologic mechanisms.
Decreased inspired oxygen pressure
Hypoventilation
• Impaired diffusion
• Ventilation/perfusion mismatch
• Shunt
Low mixed venous oxygen content
• Multiple pathophysiologic mechanisms may be at play in a single hypoxemic patient at any given time.
• However, while all six mechanisms can contribute, typically, the only clinically significant mechanisms are ventilation/perfusion mismatch (V/Q mismatch), right-to-left shunting (shunt), and low mixed venous oxygen content.
Decreased Inspired Oxygen Pressure
• In conditions of low atmospheric pressure (Patm), a decreased partial pressure of inspired oxygen (PiO2) occurs.
• PiO2 is calculated as: PiO2 = FiO2 (Patm – PH2O)
• Therefore, while the FiO2 (fraction of inspired oxygen) in the atmosphere is a constant 21%, the PiO2, and thus the driving pressure for oxygen diffusion across the alveolar membrane, is reduced in circumstances where atmospheric pressure is decreased (i.e., at altitude).
Hypoventilation
• With a reduction in alveolar ventilation, partial pressure of arterial carbon dioxide (PaCO2) will increase.
• Using the alveolar gas equation we can predict that with the increased CO2 resulting from hypoventilation, the partial pressure of alveolar oxygen (PAO2) will decrease:
PAO2 = (FiO2 × [Patm – PH2O]) – (PaCO2/0.8)
• Hypoxemia related to hypoventilation can be reversed by an increase in FiO2 using supplementary oxygen, or an increase in alveolar ventilation.
Impaired Diffusion
• Diffusion of oxygen across the alveolar–capillary membrane is rarely the sole reason for hypoxemia.
• Diffusion of gas across a membrane is governed by Fick Law: Vgas + (A × D × [P1 – P2])/T
Vgas = volume of gas diffusing
A = surface area available for diffusion
• D = diffusion coefficient of the gas
• P1 – P2 = difference in partial pressures of the gas across the membrane
T = thickness of the membrane
• In healthy subjects at rest, a single red blood cell will spend ∼0.75 seconds moving through a pulmonary capillary in contact with an alveolus.
• Oxygen is a perfusion-limited gas. Therefore, the partial pressure of oxygen in the alveolus equilibrates quickly with that in the capillary. This takes ∼0.25 seconds.
• Thus, there is considerable diffusion reserve if other variables in Fick Law are compromised.
• However, in conditions where cardiac output is increased (i.e., exercise) the red blood cell spends significantly less time in contact with the alveolus. In these instances, impaired diffusion may contribute to the development of hypoxemia (e.g., interstitial lung disease).
• Impaired diffusion is characterized by a widened Alveolar–arterial (A–a) gradient and will correct with supplemental oxygen.
Ventilation/Perfusion Mismatch
• V/Q mismatch is the most common cause of hypoxemic respiratory failure, owing to the wide variety of clinical conditions in which it occurs.
• Ideally, ventilation of alveoli would perfectly match the perfusion of blood to those alveoli resulting in a V/Q ratio of 1.
• However, even in healthy subjects, differential ventilation and perfusion of the lung from apex to base is not perfectly matched. Normally, the ventilation to perfusion ratio of the entire lung is around 0.8, accounting for the physiologic A–a gradient.
• In certain disease states (see Fig. 5-1) this ratio can be altered leading to hypoxemia.1
• V/Q mismatch can vary widely depending on the disease state.
• A V/Q ratio of infinity, where alveoli are fully ventilated but have no perfusion, is referred to as dead space.
• A V/Q ratio of 0, where alveoli are perfused but have no ventilation is referred to as a shunt.
• These two extremes of V/Q mismatch are at opposite ends of a continuous spectrum that may result in hypoxemia, hypercapnia, or both.
• V/Q mismatch is characterized by a widened A–a gradient and will correct with supplemental oxygen.
Shunt
• As discussed above, a right-to-left shunt is a specific type of V/Q mismatch with a V/Q ratio of 0.
• In true shunt physiology, deoxygenated mixed venous blood will return to systemic circulation after bypassing unventilated alveoli.
• Shunts can be congenital, as occurs in developmental abnormalities of the heart and great vessels, or acquired, as occurs in diseases affecting the lungs (see Fig. 5-1).1
• Shunt is characterized by a widened A–a gradient and inability to correct with supplemental oxygen when the shunt fraction is >30%.
Low Mixed Venous Oxygen Content
• Typically, the mixed venous oxygen content of blood returning to the right side of the heart does not affect the PaO2 significantly.
• However, in the setting of shunt or V/Q mismatch, subjects may become hypoxemic secondary to low mixed venous oxygen.
• Disease states that lower mixed venous oxygen are shown in Figure 5-1.1
DIAGNOSIS
Clinical Presentation
History
• Although hypoxemia can be asymptomatic, the most frequent presenting sign of profound hypoxemia is dyspnea.
• Copious airway secretions and abundant expectoration (among other clinical signs) can occur in pulmonary edema.
• Cough and purulent sputum (among other clinical signs) can occur in pneumonia.
Physical Examination
Manifestations of hypoxemia include cyanosis, restlessness, confusion, anxiety, delirium, tachypnea, tachycardia, hypertension, cardiac arrhythmias, and tremor.
• All of these clinical signs are both insensitive and nonspecific.
Diagnostic Criteria
Hypoxemia is defined as: PaO2 <60 mm Hg and/or (arterial oxygen saturation) SaO2 <90%.
Diagnostic Testing
• Initial diagnostic testing will likely include pulse oximetry, CXR, and arterial blood gas (ABG).
• Further diagnostic testing will depend on the differential diagnosis.
Laboratories
• A standard complete blood count (CBC) should be obtained as changes in hemoglobin can alter oxygen delivery.
• A drug screen (serum or urine) can assist in some cases of hypoventilation.
• If that patient is believed to have acute respiratory distress syndrome (ARDS), it is prudent to obtain more specific labs to diagnose the cause.
• Cultures (blood, sputum, and urine) should always be obtained if the possibility of infection is entertained.
• If diffuse alveolar hemorrhage is suspected, a vasculitis work-up would consist of antineutrophil cytoplasmic antibody (ANCA), antinuclear antibody (ANA), antiglomerular basement membrane (anti-GBM), rheumatoid factor (RF), complement levels, cryoglobulins, creatine phosphokinase (CPK), and other pertinent testing.
Imaging
• CXR: a standard two-view posterior–anterior (PA) and lateral CXR is always preferred to a portable CXR and is more sensitive for detecting and characterizing underlying lung pathology. However, the clinical situation must be taken into account.
• CT chest: depending on the clinical situation, a CT of the chest can help narrow the differential diagnosis for hypoxemic respiratory failure. A noncontrast CT scan almost always suffices, with the exception of suspicion for pulmonary embolus in which case a CT pulmonary angiogram is indicated.
Diagnostic Procedures
Echocardiogram: a cardiac echo may assist in evaluation of some causes of hypoxemia (e.g., heart failure, intracardiac right-to-left shunting, and valvular or pericardial disease).
TREATMENT
• Treatment of acute respiratory failure involves treatment of the underlying cause as well as general and respiratory supportive care.
• The therapeutic goal is to ensure adequate oxygenation of vital organs.
• For conscious, spontaneously breathing patients, supplemental oxygen is one of the most important initial treatments for acute hypoxic respiratory failure.
• The inspired oxygen concentration should be the lowest amount of supplemental oxygen that results in a PaO2 ≥60 mm Hg or a SaO2 ≥92%. Higher arterial oxygen tensions are of no proven benefit.
An important distinction must be made between adequate blood oxygenation and tissue oxygenation.
There is poor correlation between arterial hypoxemia and tissue hypoxia.
• Improvement in arterial oxygenation may not provide a subsequent or adequate improvement in tissue oxygen availability owing to a drop in cardiac output or systemic vasoconstriction.
• Obtaining a mixed venous oxygen saturation (SvO2) or central venous oxygen saturation (ScvO2) can assist with further management.
A profound decrease in tissue oxygenation is defined as shock.
• Appropriate oxygen delivery generally involves slowly increasing the inspired oxygen concentration (FiO2) with monitoring of both PaO2 and PaCO2.
• The FiO2 should slowly be increased to a goal PaO2 of 60–70 mm Hg or a SaO2 of ≥92%.
• It is important to realize that in some patients with chronic obstructive pulmonary disease (COPD) with acute-on-chronic respiratory failure there exists a compensated chronic respiratory acidosis with a small derangement of arterial pH.
• Although there is concern for CO2 retention (and resultant worsening respiratory acidosis) in COPD patients when oxygen is administered, these patients are at greater risk from acute hypoxemia than hypercapnia.
• Therefore, the goals of oxygen therapy in COPD patients are the same as for other subjects, although some sources advocate for using a SaO2 of ≥88% in these cases.
Oxygen Delivery Devices
• The selection of the appropriate noninvasive delivery system depends on the stability of the patient, availability of devices, and level of respiratory support required.
• For the critically ill or unstable patient, a planned, controlled intubation is always more desirable than emergent intubation. Predefined criteria for intubation and mechanical ventilation are broad and nonspecific, and are not covered in this chapter.
• Oxygen delivery devices for hypoxic respiratory failure can broadly be divided into two classes.
Low-flow devices, for example, nasal cannulas and face masks
High-flow devices, for example, Venturi masks and high-flow nasal cannulas
Low-Flow Oxygen Delivery Devices
Low-flow oxygen delivery devices provide a variable FiO2 based on the size of the oxygen reservoir, the rate at which the reservoir is filled, and the ventilatory pattern of the patient.
Nasal Cannulas
• These low-flow systems provide insufficient gas to replace an entire inspired tidal volume (Vt). As a result, a large part of each inhaled breath is composed of ambient (room air) gas.
• Nasal cannulas are appropriate in patients with minimal or no respiratory distress, or those who are unable to tolerate a facemask.
• The benefits include allowing the patient to eat, drink, and speak.
• Their main disadvantage is that the exact FiO2 is unknown. This is because the oxygen flow rate, the patient’s inspiratory flow rate, and the inhaled Vt all influence the final FiO2.
• As a general rule, for every liter per minute delivered, the oxygen concentration increases by ∼3%. Therefore, with a normal Vt, the commonly utilized nasal cannula flow rates of 1–6 L/min provide an FiO2 of 24–40%.
• Routine humidification of oxygen may provide little or no benefit in reducing the drying effects of nasal cannula oxygen on the nose and throat, especially with flow rates >5 L/min.
• At rates >6 L/min, flows become turbulent and the oxygen being delivered is no more effective than that delivered at 6 L/min.
Reservoir Nasal Cannulas
• Reservoir nasal cannulas are also called oxymizers.
• These low-flow systems can either increase the percentage of oxygen delivered compared to nasal cannulas, or act as oxygen-conserving devices.
• There are two types of reservoir cannulas commonly available: a nasal cannula with a reservoir situated below the nose (mustache-type), and a nasal cannula with a pendant reservoir situated on the patient’s chest (pendant-type).
• The reservoirs facilitate conservation of oxygen by storing oxygen from exhalation for delivery during subsequent inhalation.
• The reservoirs trap the initial portion of expired gas from the large conducting airways that contain a high percentage of oxygen (i.e., dead-space gas that did not participate in gas exchange in the alveoli).
• The reservoirs also receive a continuous flow of oxygen from the oxygen source.
• The combination of oxygen from the source and the reservoir results in a higher FiO2 during the subsequent inspiration and allows for total flow rates to be reduced.
Simple Facemasks
• These low-flow systems also provide a means of delivering a higher FiO2 than can be achieved via nasal cannula.
• Two types of facemask exist: those with and those without an oxygen reservoir.
• To avoid accumulation of expired air within the mask, and subsequent retention of CO2, the oxygen flow rates should be >5–6 L/min.
• Simple facemasks generally provide a FiO2 of 35–60%.
High-Flow Oxygen Delivery Devices
High-flow oxygen delivery devices provide a constant FiO2 by delivering either a higher-flow rate of oxygen than the patient’s peak inspiratory flow rate, and/or by providing an oxygen flow admixed with a fixed proportion of room air.
High-Flow Nasal Cannulas
• These high-flow systems (e.g., Optiflo) allow oxygen to be delivered at rates that exceed a patient’s inspiratory flow rate.
• Using special tubing, along with warming and humidification, allows for nasal delivery of oxygen flows of >60 L/min.
• This allows for the elimination of room air during inspiration so that a patient can breathe high fractions of oxygen without dilution by ambient air. Fractions of oxygen of up to 100% can be effectively delivered.
• In addition, the high flows used result in positive airway pressures of between 3 and 7 cm H2O that assists in preventing atelectasis.
• The main benefit of the high-flow nasal cannula over other high-flow systems is patient comfort.
Venturi Masks
• These high-flow systems deliver precise oxygen concentrations.
• Oxygen passes through a narrow orifice under pressure into a larger tube, creating a subatmospheric pressure. This drop in pressure results in a shearing force that draws room air into the delivery system through a number of openings (entrainment ports) in the tube.
• Oxygen concentration is adjusted by changing both the size of the entrainment ports and the oxygen flow.
• The maximum FiO2 achievable is 50%.
• This type of mask provides a constant FiO2 independent of changes in inspiratory flow rate.
• It allows for easy step-wise increases or decreases in FiO2 as oxygen delivery is titrated to PaO2 or SaO2.
• The main disadvantage is that the FiO2 provided by these masks is limited and may be insufficient to maintain appropriate oxygen saturations in sicker patients.
Nonrebreather Facemasks
• These high-flow systems consist of a facemask that provides a constant flow of oxygen into an attached reservoir bag, resulting in a FiO2 of >60% at 6 L/min oxygen flow.
• Each liter per minute of flow over 6 L/min increases the inspired oxygen concentration by around 10%.
• Placed correctly, the oxygen concentration can reach almost 100%.
• This type of mask is most appropriate for spontaneously breathing patients who require the highest possible oxygen concentration.
• The disadvantages of nonrebreather facemasks include oxygen toxicity, inability to feed patients owing to the tight seal required, limitation of speech, patient discomfort, and inability to provide aerosolized treatments.
Partial Rebreather Facemasks
• These high-flow systems are similar to nonrebreather facemasks.
• They differ in that they allow exhaled air to enter the reservoir, although this air is mainly from the large conducting airways and is therefore high in oxygen.
• Using the masks, a FiO2 of between 40% and 70% is achievable.
Other Therapies
• Incentive spirometry assists patients with deep breathing.
• The deep inspiration is believed to help prevent the development of significant atelectasis.
• In the setting of elective surgery, it should be started prior to the operative procedure.
• The incentive spirometer should be used >10× per hour.
• Mobilization and ambulation, through both maintenance of an upright position and exercise, may help prevent atelectasis.
MONITORING, PATIENT EDUCATION, AND FOLLOW-UP
• Oxygen should continually be weaned to a goal PaO2 of 60–70 mm Hg or a goal SaO2 of ≥92% while the patient is hospitalized and treated for their underlying ailment.
• Patients should be educated on their specific disease and what to expect in relation to long-term oxygen therapy if needed.
• Long-term oxygen therapy is indicated in patients with a PaO2 ≤55 mm Hg or SaO2 ≤88%. However, this recommendation is based on patients with COPD and may not apply to all patients with hypoxemic respiratory failure.
• Prior to discharge, a patient should be assessed for a requirement for long-term oxygen therapy both at rest and with exertion since these requirements often differ.
• A 6-minute walk can provide this information by allowing for titration of oxygen at rest and with exercise. Oxygen can then be prescribed as needed.
• If chronic oxygen needs to be administered as an outpatient, patients should be educated on the use of long-term oxygen, and the dangers associated with oxygen administration (specifically the harms of smoking or cooking with open flames when oxygen is present).
• Concentrated sources of oxygen promote rapid combustion, and when exposed to open flames highly concentrated oxygen can result in explosions.
• The amount of oxygen required to maintain oxygen saturations ≥88% can vary over time.
• Patients discharged on oxygen should be followed in the outpatient setting within 2–6 weeks depending on the etiology of their hypoxemic respiratory failure and their oxygen requirements on discharge.
• In patients requiring ongoing oxygen therapy, a 6-minute walk should be performed at least yearly to ensure an adequate oxygen prescription.
• In certain cases an ABG may be clinically indicated at follow-up.
REFERENCE
1. Kollef M, Isakow W: The Washington Manual of Critical Care. 2nd ed. Philadelphia, PA: Lippincott Williams & Wilkins; 2012.
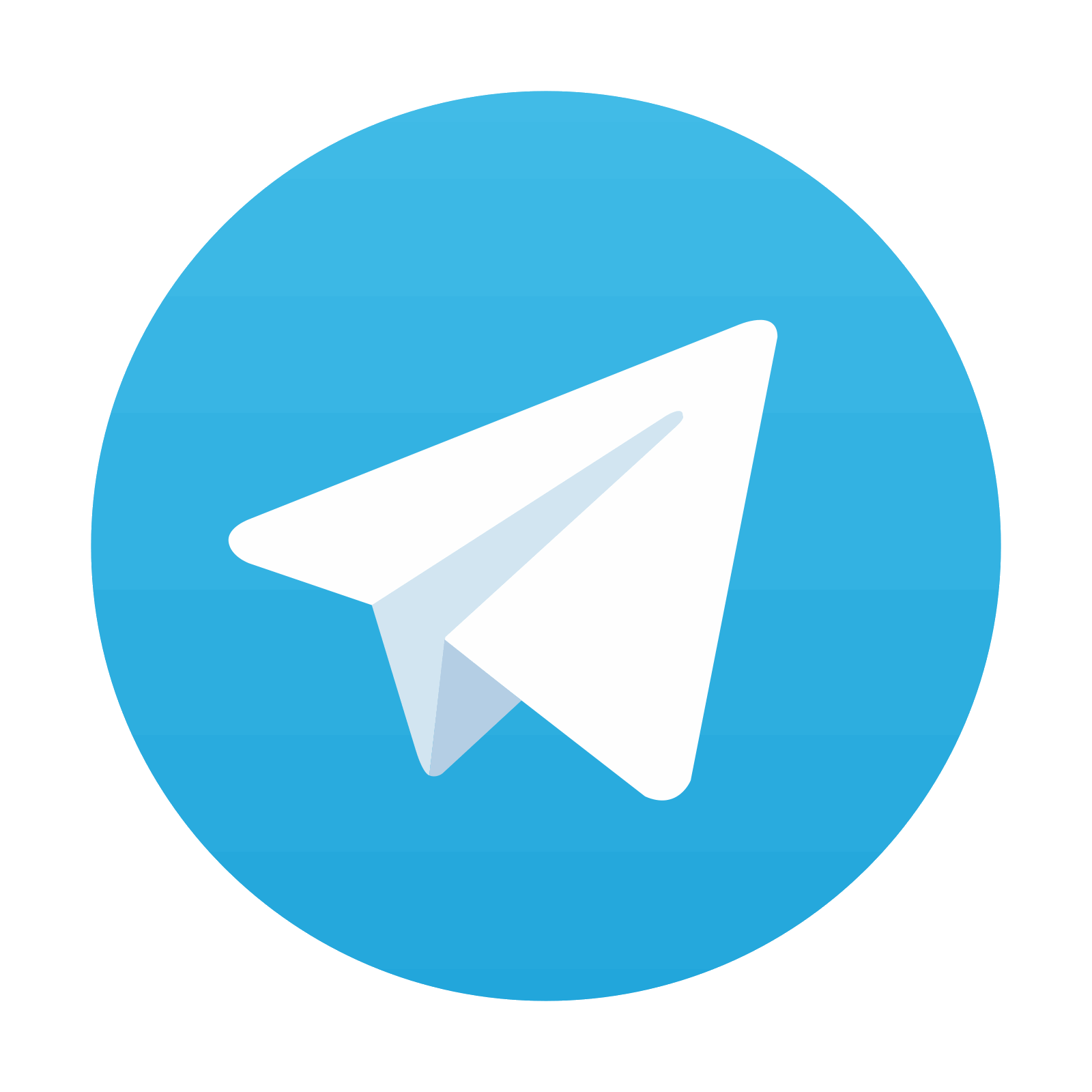
Stay updated, free articles. Join our Telegram channel
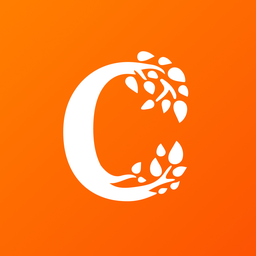
Full access? Get Clinical Tree
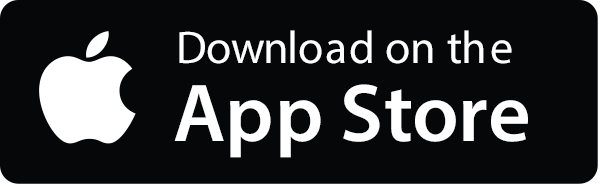
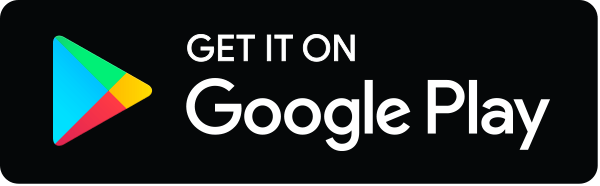