Abstract
Hypoplastic left heart syndrome constitutes a spectrum of morphologic abnormalities characterized by underdevelopment of left-sided cardiac structures. A comprehensive understanding of their unique anatomy and single-ventricle physiology is essential for successful management. Optimal outcomes are achieved by high-performing, multidisciplinary teams engaged during the fetal, preoperative, intraoperative, and postoperative periods. Significant improvement in care has been realized, but much work lies ahead as these patients continue to present challenges over their entire life span.
Key Words
Hypoplastic left heart syndrome, Norwood procedure, Stage I palliation, Single ventricle
Definition
Hypoplastic left heart syndrome (HLHS) is the term used to describe a spectrum of congenital cardiac malformations that exhibit varying degrees of underdevelopment of the left-sided heart structures. Features of HLHS include mitral valve and aortic valve atresia or stenosis, with resultant hypoplasia or absence of the left ventricle and hypoplasia of the ascending aorta and aortic arch ( Fig. 66.1 ). These anatomic defects result in single-ventricle physiology, which requires complete intracardiac mixing of pulmonary venous and systemic venous blood that is then supplied to parallel pulmonary and systemic circuits. Systemic blood flow is maintained by the right ventricle via the pulmonary artery (PA) and a patent ductus arteriosus ( Fig. 66.2 ).


This chapter focuses on the initial stage of reconstructive surgery as a treatment modality for children born with HLHS. Beyond initial reconstruction these infants are managed in a manner analogous to the management of other univentricular heart malformations destined for a modified Fontan operation. Thus considerations relevant to subsequent stages of the reconstructive sequence culminating in Fontan circulation are discussed in Chapter 63 . The other surgical option, cardiac transplantation, is discussed in detail in Chapter 73 .
Epidemiology and Embryology
HLHS is the most common congenital cardiac malformation in which only one developed ventricle is found. The syndrome was described in 1952 by Lev and later termed HLHS by Noonan and Nadas. HLHS is estimated to occur in 2.3 per 10,000 live births in the United States each year.
It accounts for 10.9% of mortalities from congenital heart disease, with a reported postoperative hospital mortality of 16% and interstage mortality of 8% to 12%. The embryologic cause of HLHS is not fully understood, but the collection of lesions likely results from limitation of left ventricular inflow or outflow.
Risk Factors
HLHS occurs predominantly in males. It may be related to genetic abnormalities and is more likely to occur in infants with a family history of congenital heart disease. Additional modifiable risk factors that have been associated with HLHS are maternal prepregnancy overweight/obesity and periconceptional opioid use or fever. Genetic syndromes in which HLHS has been seen include Turner syndrome, trisomy 13, trisomy 18, Holt-Oram, Smith-Lemli-Opitz, partial trisomy 9, and Jacobsen syndrome.
Numerous risk factors for mortality in patients with HLHS have been reported, including low birth weight, prematurity, extracardiac anomalies, and genetic syndromes. Additionally, survival may be impacted by the specific HLHS subtype, smaller ascending aorta diameter, restrictive atrial septum, associated cardiac anomalies, right ventricular dysfunction, and severity of tricuspid regurgitation (TR). Some series have reported a diminished stage I survival rate of only 79% for patients with mitral stenosis with aortic atresia, compared with the other subtypes. Furthermore, HLHS with mitral stenosis and aortic atresia is associated with ventriculocoronary connections (sinusoids), which may negatively impact outcomes and survival.
Prenatal diagnosis of patients with HLHS allows for better planning of perinatal care and is strongly associated with superior preoperative clinical status. Although prenatal diagnosis may not have a significant impact on mortality, it is related to an improvement in morbidity, specifically with regard to neurodevelopmental outcomes. Neonates who are not prenatally diagnosed are more likely to present in a shock state requiring resuscitation and inotropic support, which further increases their risk for right ventricular dysfunction and TR. Prenatal diagnosis also affords the opportunity to perform fetal interventions such as balloon dilation of the aortic valve or atrial septostomy, which may improve outcomes. Furthermore, if an intact or restrictive atrial septum is present, plans can be made for prompt balloon atrial septostomy immediately following delivery. HLHS with intact atrial septum or highly restrictive atrial septum without a decompressing vein, has significantly poorer outcomes with a survival rate of only approximately 70% to hospital discharge after the Norwood procedure.
Pathophysiology
Overview
The left ventricle is a nonfunctional structure in the child with HLHS ( Fig. 66.3 ). Pulmonary venous return must be routed to the right atrium through a stretched foramen ovale, an atrial septal defect, or rarely by total anomalous pulmonary venous connection. Systemic and pulmonary venous returns mix in the right atrium. The right ventricle supplies both the systemic and pulmonary circulations in a parallel fashion because the main PA (MPA) gives rise to the branch pulmonary arteries, as well as the systemic circulation via the ductus arteriosus. Blood flows retrograde from the ductus arteriosus through the transverse aortic arch to its branches and through the ascending aorta to the coronary arteries. Flow to the lower body is supplied antegrade from the ductus arteriosus to the descending aorta. Ductal closure results in inadequate systemic and coronary perfusion, leading to progressive metabolic acidosis, ischemia, and death. Based on this physiology, the clinical presenting signs of HLHS are (1) shock related to closure of the ductus arteriosus or (2) an imbalance in the ratio of pulmonary to systemic blood flow (Q p :Q s ), resulting in signs of congestive heart failure ( Chapter 72 ) or hypoxemia.

Changes in Pulmonary Vascular Resistance
With the pulmonary and systemic arteries connected in parallel the Q p :Q s depends on a delicate balance between the pulmonary and the systemic vascular resistances. The pulmonary vascular resistance (PVR) in any normal newborn is elevated at birth but then precipitously decreases with the first breaths and continues to fall over the first few weeks of life. The decrease in PVR results in increased, likely excessive, pulmonary blood flow. This increases the volume load of the right ventricle, and thus increased total cardiac output by the right ventricle is necessary to preserve adequate systemic output. By this mechanism, HLHS represents the most common cause of congestive heart failure in the first weeks of life. Unless other anatomic features act to limit pulmonary blood flow, the progressive imbalance in Q p :Q s will result in high-output failure, acidemia reflecting inadequate systemic perfusion, and potentially death.
Degree of Interatrial Communication
The character of the interatrial communication serves as the most common anatomic determinant of pulmonary blood flow. Because the left ventricle accepts minimal or no flow, the interatrial communication provides the only route for egress of pulmonary venous blood entering the left atrium. In neonates with HLHS and a foramen ovale as the only interatrial communication, left atrial pressure typically exceeds right atrial pressure, so blood will flow left to right at the foramen ovale (see Fig. 66.2 ). The resultant elevation in pulmonary venous pressure increases PVR and limits pulmonary blood flow. When the foramen ovale or an additional atrial septal defect permits unobstructed interatrial flow of blood from left to right, pulmonary blood flow increases dramatically as PVR decreases. Alternatively, excessive narrowing or obliteration of the foramen ovale imposes severe restriction on pulmonary blood flow. Therefore the systemic saturation reflects the mixture of systemic venous return with variable pulmonary venous return permitted by the interatrial communication (see Fig. 66.2 ).
Diagnostic Assessment
Physical Findings
Neonates with a nearly balanced Q p :Q s will have adequate systemic perfusion, as evidenced by a normal systemic arterial blood pressure, warm extremities with good peripheral pulses, and the absence of a metabolic acidemia. The skin color is usually dusky. Auscultation reveals a systolic ejection murmur and a single second heart sound.
Patients with widely patent atrial septal defects have a Q p :Q s well in excess of 1 : 1. This can be tolerated as long as the right ventricle can maintain a compensatory increase in the total cardiac output (total Q). However, if total Q is inadequate, excessive pulmonary blood flow occurs at the expense of systemic blood flow, and systemic hypoperfusion develops. The physical findings in this cohort are dominated by evidence of shock and congestive heart failure. These infants appear listless and tachypneic with diminished pulses in all extremities. The liver is enlarged. Acidosis, hyperkalemia, and hypoglycemia signify metabolic decompensation.
Conversely, patients with little or no interatrial communication are deeply cyanotic because of inadequate pulmonary blood flow. Although this cohort may appear vigorous immediately after delivery, the deleterious cardiovascular and metabolic effects of profound hypoxemia, characterized by PaO 2 values of 20 mm Hg or lower in the most severe cases, ultimately become apparent. Myocardial performance deteriorates, and the infant will exhibit listlessness, diminished peripheral pulses, acidosis, and hypoglycemia.
Diagnostic Tests
The electrocardiogram (ECG) shows right atrial enlargement with peaked P waves in leads II, III, and aVF and right ventricular enlargement with a qR pattern in the right precordial leads. The chest radiograph reveals cardiomegaly because of right atrial, right ventricular, and proximal PA enlargement. The lung fields exhibit pulmonary congestion in the majority. In patients with severely obstructed interatrial communication the cardiomegaly is less prominent, and the pulmonary vascular markings vary from diminished to congested in a more reticular pattern, which reflects the pulmonary venous hypertension.
Two-dimensional echocardiography is sufficient to diagnose this lesion. Echocardiographic imaging determines the anatomic details. Pulse and continuous wave Doppler in conjunction with color flow analysis are then used to evaluate several aspects of the physiology. For example, the relative pulmonary and systemic resistances can be inferred from the direction of flow in the ductus arteriosus during diastole. Assessment of the tricuspid or common atrioventricular valve for regurgitation is particularly important, as well as identification of the insertion and drainage of the pulmonary veins.
Routine cardiac catheterization is not necessary in the evaluation of neonates with HLHS.
Preoperative Critical Care Management
All interventions in the preoperative period are directed toward two goals: (1) preserving ductal patency and (2) establishing or maintaining the Q p :Q s ratio at unity.
Prostaglandin E 1 Infusion
Nearly all infants will have physiologic closure of the ductus arteriosus by the fourth day of life, but 20% of infants will demonstrate functional ductal closure during the first day of life, and more than 80% of infants demonstrate ductal closure during the second day of life. A continuous infusion of prostaglandin E 1 (PGE 1 ) should be instituted once the diagnosis of HLHS has been made to preserve the infant’s ductal dependent systemic circulation. Patients who were not diagnosed prenatally should receive PGE 1 infusion as soon as the diagnosis is suspected. Hence any newborn with unexplained shock is a candidate for PGE 1 infusion until HLHS or other forms of ductal dependent systemic circulation have been specifically excluded.
For patients who present in shock with suspected ductal closure or a restrictive ductus, initial prostaglandin dosing will range from 0.05 to 0.1 mcg/kg/min, and then once ductal patency is achieved, the infusion can be decreased to an effective dose of 0.01 to 0.02 mcg/kg/min. It is important to maintain ductal patency with the lowest effective prostaglandin dose to minimize the most common dose-dependent side effects of the medication. Hypotension requiring volume resuscitation and respiratory depression requiring mechanical ventilator support may occur.
Physiologic Assessment and Manipulation of the Ratio of Pulmonary Blood Flow to Systemic Output
Much of the preoperative management of neonates with HLHS entails optimizing the condition of the cardiovascular and other organ systems. Because the right ventricle must perfuse the pulmonary and systemic circulations in parallel, emphasis is placed on strategies that promote optimal systemic oxygen delivery with minimal overall volume work imposed on the ventricle. This goal is usually attained when pulmonary blood flow approximates systemic output (i.e., Q p :Q s is 1 : 1). Although HLHS is not unique in posing the need to balance Q p :Q s , there is a tendency for this cardiac malformation to exhibit high pulmonary blood flow and marginal systemic output. Perhaps the anatomic relation whereby the systemic flow must traverse the MPA on its way to the aorta contributes to this phenomenon. In addition, speculation persists as to whether the right ventricle is intrinsically less well suited to the excess volume work imposed by a high Q p :Q s state.
The key to management of HLHS perioperatively rests with the ability to assess systemic perfusion and Q p :Q s . These variables often cannot be quantified precisely under typical clinical circumstances, but clinicians can predict, measure, and manipulate this complex physiology by calculating Q p :Q s according to the Fick equation:
Q p : Q s = ( Ao − SVC ) / ( PV − PA )
In patients with cardiac lesions that result in complete mixing and parallel systemic and pulmonary circulations, one can assume that content in the aorta and PA are equal, and the former is usually conveniently measured. When significant pulmonary disease does not complicate the clinical situation, clinicians typically assume that the PV is fully saturated with oxygen, or nearly so. That leaves the SVC content to be ascertained, and in the clinical environment, measurement of oxygen saturation usually replaces calculation of content because accurate measurement instruments are readily available, and the relation of the two values is reasonably constant. SVC saturation can be directly measured by obtaining a mixed venous saturation from a central line residing in the SVC or indirectly measured by near-infrared spectroscopy (NIRS) monitoring, a noninvasive way to continuously trend the patient’s mixed venous oxygen saturation. NIRS values decrease as oxygen delivery decreases or demand increases and have been shown to assist with detection of hypoxic-ischemic conditions.
Without a mixed venous oxygen saturation measurement, the clinical estimate of Q p :Q s is made on the basis of a single value, the systemic arterial saturation. However, it is problematic to assume that systemic venous saturation, as well as the difference between arterial oxygen saturation and mixed venous oxygen saturation (AVO 2 difference), is normal, especially given that the physiology of these infants makes it highly likely that systemic cardiac output may be inadequate.
For example, if the systemic oxygen saturation were 80%, and normal values are assumed for pulmonary venous saturation and AVO 2 difference, the calculation would result as follows:
Q p : Q s = ( 80 − 60 ) / ( 100 − 80 ) = 1
By using similar assumptions and a systemic oxygen saturation of 60%, the Q p :Q s calculation would result as follows:
Q p : Q s = ( 60 − 40 ) / ( 100 − 60 ) = 0.5
A systemic oxygen saturation of 90% might yield the following:
Q p : Q s = ( 90 − 70 ) / ( 100 − 90 ) = 2
However, these calculations can be highly inaccurate when such assumptions are made. The calculated values can be significantly altered by changing the underlying assumptions, even within normal boundaries.
For example, an infant with HLHS and excessive pulmonary blood flow at the expense of systemic perfusion could have a decreased mixed venous saturation. Using a directly or indirectly measured value for mixed venous saturation, the calculation might result as follows:
Q p : Q s = ( 90 − 50 ) / ( 100 − 90 ) = 4
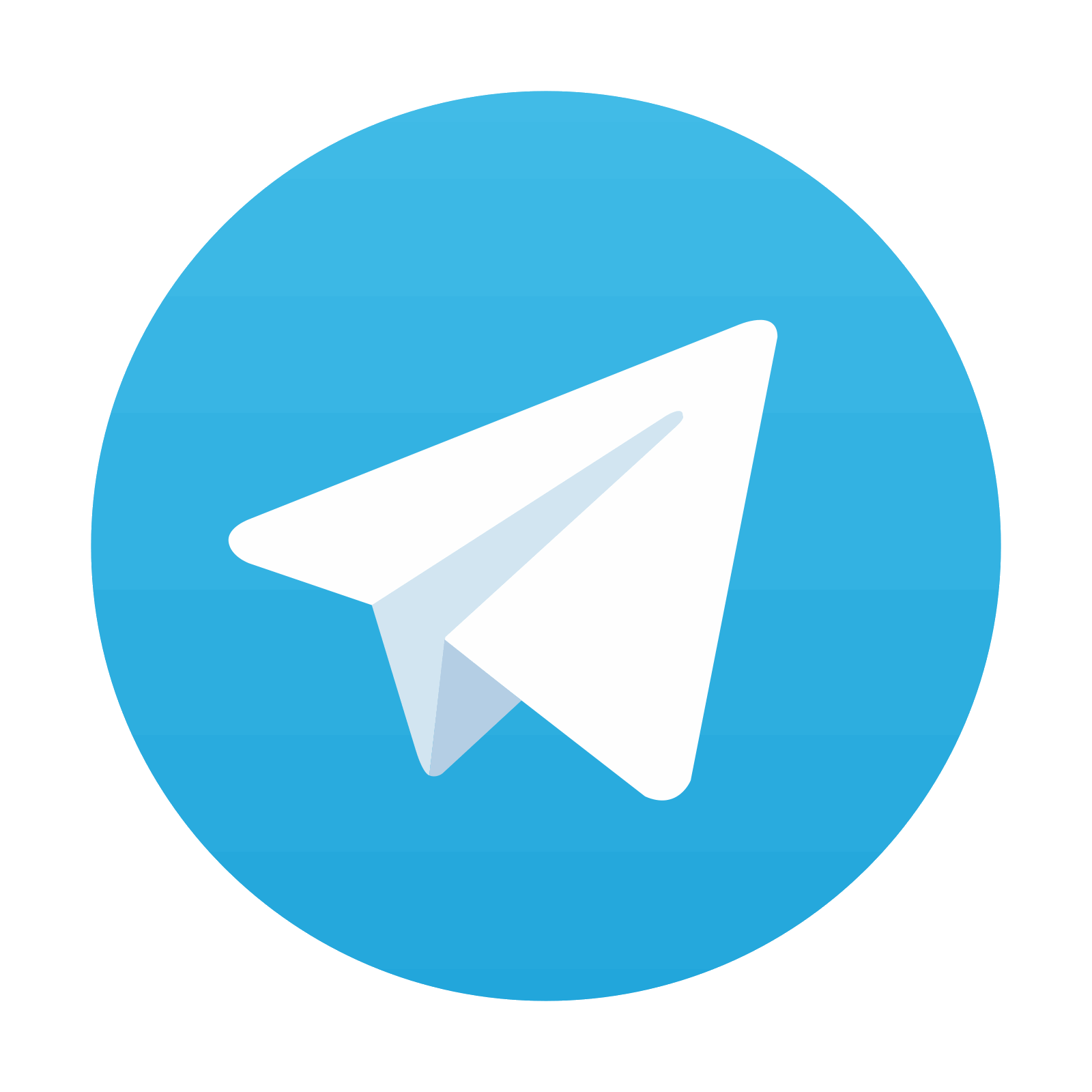
Stay updated, free articles. Join our Telegram channel
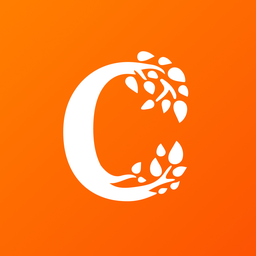
Full access? Get Clinical Tree
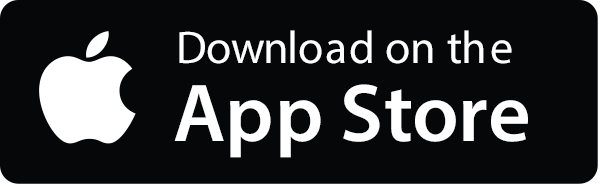
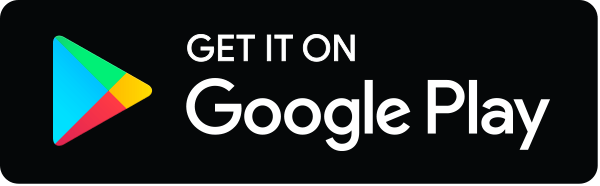