Chapter 26 Hyperoxia and oxygen toxicity




Chapter 24 described the disastrous consequences of lack of oxygen for life forms that depend on it, but for most organisms hypoxia is an infrequent event. However, oxygen itself also has toxic effects at the cellular level, which organisms have had to oppose by the development of complex antioxidant systems. The activity of toxic oxygen derivatives and antioxidant systems is perfectly balanced for most of the time. Nevertheless, there is a strengthening opinion that over many years oxidative mechanisms predominate, and may be responsible for the generalised deterioration in function associated with ageing.1 In a variety of diseases, or when exposed to extra oxygen, the balance is radically disturbed and oxidative tissue damage results.
Hyperoxia
Hyperventilation, while breathing air, can raise the arterial Po2 to about 16 kPa (120 mmHg). Higher levels can be obtained only by oxygen enrichment of the inspired gas and/or by elevation of the ambient pressure. Although the arterial Po2 can be raised to very high levels, the increase in arterial oxygen content is usually relatively small (Table 26.1). The arterial oxygen saturation is normally close to 95% and, apart from raising saturation to 100%, additional oxygen can be carried only in physical solution. Provided that the arterial/mixed venous oxygen content difference remains constant, it follows that venous oxygen content will rise by the same value as the arterial oxygen content. The consequences in terms of venous Po2 (Table 26.1) are important because minimum tissue Po2 approximates more closely to venous than to arterial Po2. The rise in venous Po2 is trivial when breathing 100% oxygen at normal barometric pressure, and it is necessary to breathe oxygen at 3 atmospheres absolute (ATA) pressure before there is a large increase in venous and therefore tissue Po2. This is because most of the body requirement can then be met by dissolved oxygen, and the saturation of capillary and venous blood remains close to 100%.
Table 26.1 Oxygen levels attained in the normal subject by changes in the oxygen tension of the inspired gas
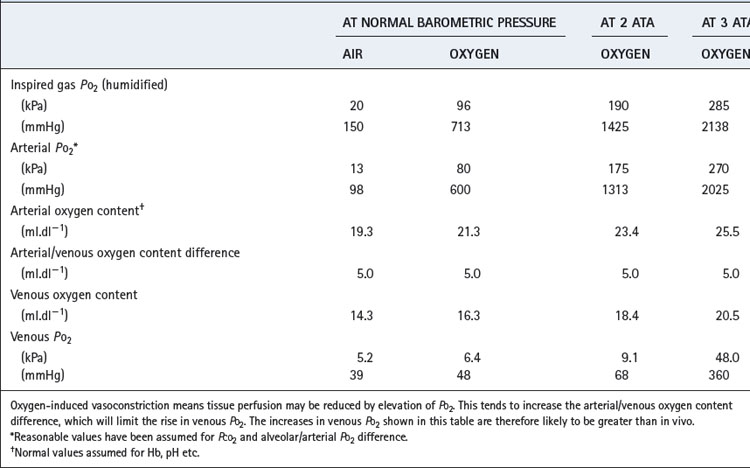
It is convenient to consider two degrees of hyperoxia. The first applies to the inhalation of oxygen-enriched gas at normal pressure, while the second involves inhaling oxygen at raised pressure and is termed hyperbaric oxygenation.
Hyperoxia at Normal Atmospheric Pressure
The commonest indications for oxygen enrichment of the inspired gas are the prevention of arterial hypoxaemia (‘anoxic anoxia’) caused either by hypoventilation (page 399) or by venous admixture (page 133). Oxygen enrichment of the inspired gas may also be used to mitigate the effects of hypoperfusion (‘stagnant hypoxia’). The data in Table 26.1 show that there will be only marginal improvement in oxygen delivery (page 202), but it may be critical in certain situations. ‘Anaemic anoxia’ will be only partially relieved by oxygen therapy but, because the combined oxygen is less than in a subject with normal haemoglobin concentration, the effect of additional oxygen carried in solution will be relatively more important.
Clearance of gas loculi in the body may be greatly accelerated by the inhalation of oxygen, which greatly reduces the total tension of the dissolved gases in the venous blood (Table 26.2). This results in the capillary blood having additional capacity to carry away gas dissolved from the loculi. Total gas tensions in venous blood are always slightly less than atmospheric, and this is of critical importance in preventing the accumulation of air in potential spaces such as the pleural cavity, where the pressure is subatmospheric. Oxygen is therefore useful in the treatment of air embolism (page 426) and pneumothorax (page 446).
Hyperbaric Oxygenation
Mechanisms of Benefit
Effect on Po2. Hyperbaric oxygenation is the only means by which arterial Po2 values in excess of 90 kPa (675 mmHg) may be obtained. However, it is easy to be deluded into thinking that the tissues will be exposed to a similar Po2 as found in the chamber. Terms such as ‘drenching the tissues with oxygen’ have been used but are meaningless. In fact, the simple calculations shown in Table 26.1, supported by experimental observations, show that large increases in venous and presumably therefore minimum tissue Po2 do not occur until the Po2 of the arterial blood is of the order of 270 kPa (2025 mmHg), when the whole of the tissue oxygen requirements can be met from the dissolved oxygen. However, the relationship between arterial and tissue Po2 is highly variable (page 155), and hyperoxia-induced vasoconstriction in the brain and other tissues limits the rise in venous and tissue Po2. Direct access of ambient oxygen will increase Po2 in superficial tissues, particularly when the skin is breached.
Angiogenesis. The growth of new blood vessels is improved when oxygen is increased to more than 1 ATA pressure.2 There seems to be no effect with 100% oxygen at 1 ATA,3 and the mechanism by which angiogenesis is promoted is uncertain. When normoxia follows a period of hypoxia, reactive oxygen species (see below) are produced, and these are known to stimulate the production of a variety of growth factors that initiate angiogenesis.4 The same mechanism may occur during hyperbaric oxygenation.5
Anti-bacterial effect. For many years oxygen was believed to play a role in bacterial killing by the formation of reactive oxygen species, particularly in polymorphs and macrophages, though this has recently been refuted (see below). However, oxygen will still have a direct toxic effect on micro-organisms, particularly on anaerobic bacteria, and relief of hypoxia improves the performance of polymorphs.6
Boyle’s Law effect. The volume of gas spaces within the body is reduced inversely to the absolute pressure according to Boyle’s Law (page 515). This effect is additional to that resulting from reduction of the total tension of gases in venous blood (see above).
Clinical Applications of Hyperbaric Oxygenation7
Gas embolus and decompression sickness are unequivocal indications for hyperbaric therapy and the rationale of treatment is considered above and in Chapter 18.
Carbon monoxide poisoning. In spite of the exploitation of natural gas, there remains a high incidence of carbon monoxide poisoning from automobile exhausts, fires and defective domestic heating appliances. Indications for hyperbaric oxygenation following carbon monoxide poisoning include age over 36 years, loss of consciousness or carboxyhaemoglobin (COHb) levels of more than 25%,8 and it is now accepted that therapy improves delayed neurological sequelae.7,9,10 The rationale of therapy – increased rate of dissociation of COHb – seems simple when the half-life of COHb is approximately 4–5 hours whilst breathing air and only 20 minutes with hyperbaric oxygen. However, breathing 100% oxygen at normal pressure reduces the half life of COHb to just 40 minutes, and therefore in many cases, by the time transport to a hyperbaric chamber is achieved, COHb levels will already be considerably reduced. Other potential benefits of hyperbaric oxygen are believed to derive from minimising the effects of carbon monoxide on cytochrome-c oxidase and reducing lipid peroxidation and neutrophil adherence.9
Wound healing is improved by hyperbaric oxygenation, even when used intermittently. It is particularly useful when ischaemia contributes to the ineffective healing – for example, in diabetes mellitus or peripheral vascular disease. The mechanisms are similar to those for burns, and, in both cases, improved tissue oxygen levels probably result from direct diffusion of oxygen into the affected superficial tissues and increased release of growth factors.5
Sports injuries. Hyperbaric oxygen is believed to expedite recovery from soft-tissue injuries and fractures incurred during competitive sports.11 Early treatment (within 8 hours) is most effective indicating a probable effect on neutrophil activity at the site of injury.12
Multiple sclerosis. In the early 1980s there was great interest in the therapeutic value of hyperbaric oxygenation in multiple sclerosis. A small study reported a favourable response in a double-blind controlled trial in which the treated group received 2 ATA oxygen, while the placebo group inhaled 10% oxygen in nitrogen, also at 2 atmospheres.13 Unfortunately, these findings were not confirmed in subsequent studies, and a review of 14 controlled trials concluded that hyperbaric oxygen cannot be recommended for the treatment of multiple sclerosis.14
Oxygen Toxicity
The Oxygen Molecule and Reactive Oxygen Species (ROS)15,16
The dioxygen molecule (Figure 26.1) is unusual in having two unpaired electrons in the outer (2P) shell. Thus dioxygen itself qualifies as a ‘double’ free radical, but stability is conferred by the fact that the orbits of the two unpaired electrons are parallel. The two unpaired electrons also confer the property of paramagnetism, which has been exploited as a method of gas analysis that is almost specific for oxygen (page 208).
Singlet oxygen. Internal rearrangements of the unpaired electrons of dioxygen result in the formation of two highly reactive species, both known as singlet oxygen (1O2). In 1ΔgO2 one unpaired electron is transferred to the orbit of the other (Figure 26.1), imparting an energy level 22.4 kcal.mol−1 above the ground state. There being no remaining unpaired electron, 1ΔgO2 is not a ROS. In 1Σg+, the rotation of one unpaired electron is reversed, which imparts an energy level 37.5 kcal.mol−1 above the ground state and this molecule is a ROS. 1Σg+ is extremely reactive, and rapidly decays to the 1ΔgO2 form, which is particularly relevant in biological systems and especially to lipid peroxidation.
Superoxide anion. Under a wide range of circumstances, considered below, the oxygen molecule may be partially reduced by receiving a single electron, which pairs with one of the unpaired electrons forming the superoxide anion (O2− in Figure 26.1), which is both an anion and a ROS. It is the first and crucial stage in the production of a series of toxic oxygen-derived ROS and other compounds. The superoxide anion is relatively stable in aqueous solution at body pH, but has a rapid biological decay due to the ubiquitous presence of superoxide dismutase (see below). Being charged, superoxide anion does not readily cross cell membranes.
Hydrogen peroxide. Superoxide dismutase (SOD) catalyses the transfer of an electron from one molecule of the superoxide anion to another. The donor molecule becomes dioxygen while the recipient rapidly combines with two hydrogen ions to form hydrogen peroxide (Figure 26.1). Although hydrogen peroxide is not a ROS, it is a powerful and toxic oxidising agent that plays an important role in oxygen toxicity. The overall reaction is as follows:
Three stage reduction of oxygen. Figure 26.2 summarises the three-stage reduction of oxygen to water, which is the fully reduced and stable state. This contrasts with the more familiar single-stage reduction of oxygen to water that occurs in the terminal cytochrome (page 200). Unlike the single-stage reduction of oxygen, the three-stage reaction shown in Figure 26.2 is not inhibited by cyanide.