Fig. 62.1
Major cardiac ion channels and associated primary electrical disorders. Abbreviations: I current, ↑ current increase (gain-of-channel function), ↓ current decrease (loss-of-channel function), I K potassium current, I Na sodium current, I Ca calcium current, LQTS long QT syndrome, SQTS short QT syndrome, IVF idiopathic ventricular fibrillation, PFHB progressive familial heart block, ATFB atrial fibrillation, BRGDA Brugada syndrome, SSS sick sinus node syndrome, ERS early repolarization syndrome, JLNS Jervell and Lange-Nielsen syndrome, LVNC left ventricular noncompaction cardiomyopathy. Gene symbols are in italic
Table 62.1
Potassium channel genes (KCNx) and inherited forms of arrhythmias
Gene | Disease (OMIM) | Protein, current | Sensitivity | Inheritance | Channel dysfunction |
---|---|---|---|---|---|
A: >10 % | |||||
B: 1–10 % | |||||
C: <1 % | |||||
KCNA2 | BRGDA | ß-Subunit (Kvß3) | C | AD | Gain of function, Ito ↑ |
Ito | |||||
KCNA5 | ATFB7 | Major subunit | B | AD | Loss of function, IKur ↓ |
(Kv1.5) | Gain of function, IKur ↑ | ||||
IKur | |||||
KCND3 | BRGDA | Accessory subunit | C | Gain of function, Ito ↑ | |
(Kv4.3) | |||||
ATFB | Ito,f | C | Gain of function, Ito ↑ | ||
KCNQ1 | LQT1 | α-Subunit | A | AD | Loss of function, IKs ↓ |
JLN1 | A | AR | Loss of function, IKs ↓ | ||
SQT2 | (Kv7.1) | C | AD | Gain of function, IKs ↑ | |
ATFB3 | IKs | C | AD | Gain of function, IKs ↑ | |
KCNH2 | LQT2 | α-Subunit | A | AD | Loss of function, IKr ↓ |
(Kv11.1) | |||||
SQT1 | IKr | C | AD | Gain of function, IKr ↑ | |
KCNE1 | LQT5 | ß-Subunit | C | AD | Loss of function, IKs ↓ |
JLN2 | IKs/Kr | A | AR | Loss of function, IKs ↓ | |
ATFB | C | Gain of function, IKs ↑ | |||
KCNE2 | LQT6 | ß-Subunit | C | Loss of function, IK ↓ | |
ATFB4 | IK | C | Gain of function, IK ↑ | ||
KCNE3 | BRGDA6 | ß-Subunit | C | Gain of function, IK ↑ | |
ATFB | IK | C | Gainof function, IK ↑ | ||
KCNE5 (KCNE1L) | IVF | ß-Subunit | C | Gain of function, Ito ↑ | |
ATFB | IK | C | Gain of function, IKs ↑ | ||
KCNJ2 | Andersen-Tawil | Major subunit | A | AD | Gain of function, IK1 ↑ |
LQT7 | (Kir2.1) | C | AD | Gain of function, IK1 ↑ | |
SQT3 | IK1 | C | AD | Gain of function, IK1 ↑ | |
ATFB9 | C | AD | Gain of function, IK1 ↑ | ||
KCNJ5 | Andersen-Tawil | Major subunit | C | AD | Loss of function, IK,ACh ↓ |
(Kir3.4) | |||||
IK,ACh | |||||
LQT13 | C | AD | Loss of function, IK,ACh ↓ | ||
KCNJ8 | ERS, IVF | Major subunit | C | Gain of function, IK,ATP ↑ | |
(Kir6.1) | |||||
IK,ATP |
Table 62.2
Sodium channel genes (SCNx) and inherited forms of arrhythmias
Gene | Disease (OMIM) | Protein, current | Sensitivity | Inheritance | Channel dysfunction |
---|---|---|---|---|---|
A: >10 % | |||||
B: 1–10 % | |||||
C: <1 % | |||||
SCN5A | ATFB10 | α-Subunit INaV1.5 | A | AD | Loss of function, INa↓ |
Gain of function, INa↑ | |||||
BRGDA1 | A | AD | Loss of function, INa↓ | ||
CMD1E | B | AD | Loss of function, INa↓ | ||
Gain of function, INa↑ | |||||
IVF | C | Loss of function INa↓ | |||
LQT3 | B | AD | Gain of function, INa↑ | ||
PFHB1A (PFHBI) | A | AD | Loss of function, INa↓ | ||
SSS1 | B | AD, AR | Loss of function, INa↓ | ||
MEPPC | AD | Gain of function, INa↑ | |||
ATRST1 | C | ||||
SCN10A | BRGDA | α-Subunit INaV1.8 | C | AD | Loss of function, INa↓ |
SCN1B | BRGDA5 | ß-Subunit INa | C | AD | Loss of function, INa↓ |
ATFB13 | C | Loss of function, INa↓ | |||
SCN2B | BRGDA | ß-Subunit INa | C | AD | Loss of function, INa↓ |
ATFB14 | C | Loss of function, INa↓ | |||
SCN3B | IVF | ß-Subunit INa | B | Loss of function, INa↓ | |
BRGDA7 | C | Loss of function, INa↓ | |||
ATFB16 | C | Loss of function, INa↓ | |||
SCN4B | LQT10 | ß-Subunit INa | C | AD | Gain of function, INa↑ |
ATFB17 | C |
Table 62.3
Calcium channel (CACNx) and cation channel genes and inherited forms of arrhythmias
Gene | Disease (subform) | Protein | Sensitivity | Inheritance | Dysfunction |
---|---|---|---|---|---|
A: >10 % | |||||
B: 1–10 % | |||||
C: <1 % | |||||
CACNA1C | Timothy syndrome | α-Subunit CaV1.2 | A | AD | Gain of function, ICaL ↑ |
LQT8 | C | (-) | Gain of function, ICaL ↑ | ||
BRGDA3 | B | AD | Loss of function, ICaL ↓ | ||
SQT4 | C | AD | Loss of function, ICaL ↓ | ||
ERS, IVF | B | AD | Loss of function, ICaL ↓ | ||
CACNA1D | SANDD | α-Subunit CaV1.3 | C | AR | Loss of function, ICaL ↓ |
CACNA2D1 | BRGDA10 | α-,δ-Subunit | B | AD | Loss of function, ICaL ↓ |
ERS, IVF | B | AD | Loss of function, ICaL ↓ | ||
SQT6 | C | AD | Loss of function, ICaL ↓ | ||
CACNB2 | BRGDA4 | ß-Subunit | B | AD | Loss of function, ICaL ↓ |
ERS, IVF | B | AD | Loss of function, ICaL ↓ | ||
SQT5 | C | AD | Loss of function, ICaL ↓ | ||
RyR2 | CPVT1 | Ryanodine receptor 2 (RYR2) | A | AD | Gain of function, |
diastolic [Ca2+]i ↑ | |||||
CASQ2 | CPVT2 | Calsequestrin 2 | C | AR | Loss of function, |
diastolic [Ca2+]i ↑ | |||||
TRDN | CPVT5 | Triadin | C | AR | Loss of function |
HCN4 | SSS2 | Cardiac pacemaker (cation) channel | B | AD | Loss of function, If ↓ |
TRPM4 | PFHB1b | Cardiac cation channel (Purkinje cells) | A | AD | Gain of function, ↑ |
Many identified mutations are “private” (i.e., family specific). Pinpoint protein regions of the native ion channel that cause ion channel dysfunction have been identified and may allow modification by drugs. Pathophysiological and common disease pathways can be recreated in patient-derived cellular models (human-induced pluripotent stem cells; hiPSCs) and transdifferentiated cardiomyocyte-like cells that share the patient’s genetic setting and are subjected to comprehensive biomedical research.
Nearly every inherited arrhythmia is genetically heterogeneous. For some primary electrical disorders (PEDs) of the heart, more than 10 different genes or associated loci are known (e.g., long QT syndrome, atrial or ventricular fibrillation). In addition, there is significant allelic heterogeneity (e.g., > 300 different LQTS mutations). In contrast, in some PEDs the mutation detection rate (“sensitivity of a genetic test”) is still low (e.g., atrial or ventricular fibrillation, 10–20 %) raising the issue of clinical phenotypic conditions. Therefore, precise knowledge and recognition of the genetic forms of PEDs are essential, including differentiation from non-genetic forms. In the light of existing genetic heterogeneity, but also of unforeseen genetic complexity in known disease genes, next-generation sequencing (NGS) technologies will improve modern genetic diagnostics. Together with sufficient pathogenicity variant prediction, this parallelized gene analysis (e.g., several hundreds of genes for a distinct phenotype in a single analysis run) will further replace DNA analysis depending on Sanger sequencing approaches. However, apart from delineating the genomic complexity of monogenic cardiac disorders, it is likely that upon NGS analyses, cardiovascular genes not being previously linked to the patient’s phenotype will be addressed and novel genes may be identified. Importantly, additional confirmatory research steps are required to establish a relationship between a novel gene and the phenotype.
62.2 Inherited Forms of Ventricular Arrhythmias
Familial forms of arrhythmias were described carefully several decades ago [e.g., 1–6] and were essential to elucidate the genetic basis of arrhythmias. These clinical observations are still important today, since in the era of human molecular genetics, these disorders have been subject to systematic gene investigations and identification. The rapid technological improvements of molecular genetic approaches – currently as whole-exome sequencing by NGS – and the detailed knowledge of the human genome [7, 8] have replaced genetic linkage and many candidate gene analyses (see Chap. 18). NGS certainly will speed up gene identification [9–13], as, for example, has been shown for familial forms of ventricular fibrillation due to a CALM1 gene mutation [14].
62.3 Congenital Long QT Syndrome (LQTS)
LQTS is characterized by prolongation of the QT interval, typically measured in a baseline 12-lead or exercise ECG (recommended speed, 50 mm/s). These values have to be corrected for heart rate (by using Bazett’s formula to drive the corrected QTc value). A QTc of >450 ms (males) or >460 ms (females) is indicative for a LQTS but there is overlap with the normal population. The presence of interventricular conduction delay such as complete right or left bundle branch block may limit the use of the QT interval. The QT interval also can be affected by many drugs. In contrast to congenital LQTS, drug-induced QT prolongation (“acquired LQTS”) is often not genetic, and only in 10–15 % of case can the drug be considered to have unmasked “hidden LQTS” [17]. For many physicians, recognition of LQTS and accurate QT interval measurements are still difficult [18]. Due to cascade family investigations and systematic genetic testing, it now has become clear that there are many LQTS mutation carriers without symptoms [19]. These asymptomatic but LQTS mutation-positive patients still have congenital LQTS, even though the term “syndrome” might be misleading. The overall risk for cardiac events or arrest might be lower and mainly is determined by the degree of QT interval prolongation and exposure to risk or event-triggering factors [20, 21].
Since 1995, 13 genes have been associated with congenital LQTS. A significant portion of mutations (sensitivity approx. 35 %) can be identified in the KCNQ1 gene (subform LQT1; chromosome 11p15.5) encoding the α-subunit of the slowly activating, delayed outward rectifying K+ channel IKs (Kv7.1). These subunits form together with additional ß-subunits (e.g., KCNE1; LQT5) tetrameric channels. Mutations in KCNQ1 mainly result in decreased potassium outward current (“loss of function”). In addition to digenic inheritance [in 3–5 %, 22], other severe forms are the autosomal recessive Jervell and Lange-Nielsen syndrome (JLNS) with additional severe, bilateral sensorineural hearing loss. The second key gene for LQTS is the KCNH2 gene on chromosome 7q36.1 that encodes the pore-forming α-subunit of the rapidly activating delayed rectifier potassium channel IKr (Kv11.1; human ether-a-go-go-related gene (hERG)) and plays an essential role in the final repolarization of the ventricular action potential as well as in drug-induced proarrhythmia [23]. “Loss-of-function” mutations in KCNH2 (sensitivity, 30 %) lead to LQT2, whereas opposite effects by a “gain of function” shorten the QT interval (so-called short QT syndrome, SQT1). Another LQTS subform, LQT3, is related to cardiac sodium channel defects by SCN5A gene (chromosome 3p22.2) mutations. The gene encodes the α-subunit from cardiac isoform of the voltage gated sodium channel (Nav1.5); the main electrophysiological mechanism is a “gain of function” (either due to abnormal channel gating or impaired trafficking) with pronounced inward sodium (INa) current, and mutations occur in 10–15 % of LQTS patients. In addition to the molecular and mechanistic differences in the major LQTS subforms, patients differ clinically by differences in the pharmacological response, phenotypic disease modification (e.g., repolarization pattern in surface ECG), and disease severity [e.g., 20, 21].
Concerning additional but mainly rare LQTS subforms (sensitivity <1 % or less), mutations in other ion channel genes or regulatory subunits have been found. These ion channel subunits are encoded by KCNE2 (K+; LQT6), KCNJ2 (K+; LQT7; Andersen-Tawil syndrome), KCNJ5 (K+; LQT13), and SCN4B (Na+; LQT10). Other genes for LQTS, sometimes based on a well-characterized single case report with a proven functional basis of the mutant, are ANK2 (ankyrin-B, LQT4), AKAP9 (A-kinase anchor protein; LQT11), CACNA1C (Ca2+; LQT8; also Timothy syndrome), CALM1 (calmodulin 1), CALM2 (calmodulin 2), CALM3 (calmodulin 3), CAV3 (caveolin 3; LQT9), and SNTA1 (syntrophin alpha 1; LQT12) (Tables 62.1 – 62.3).
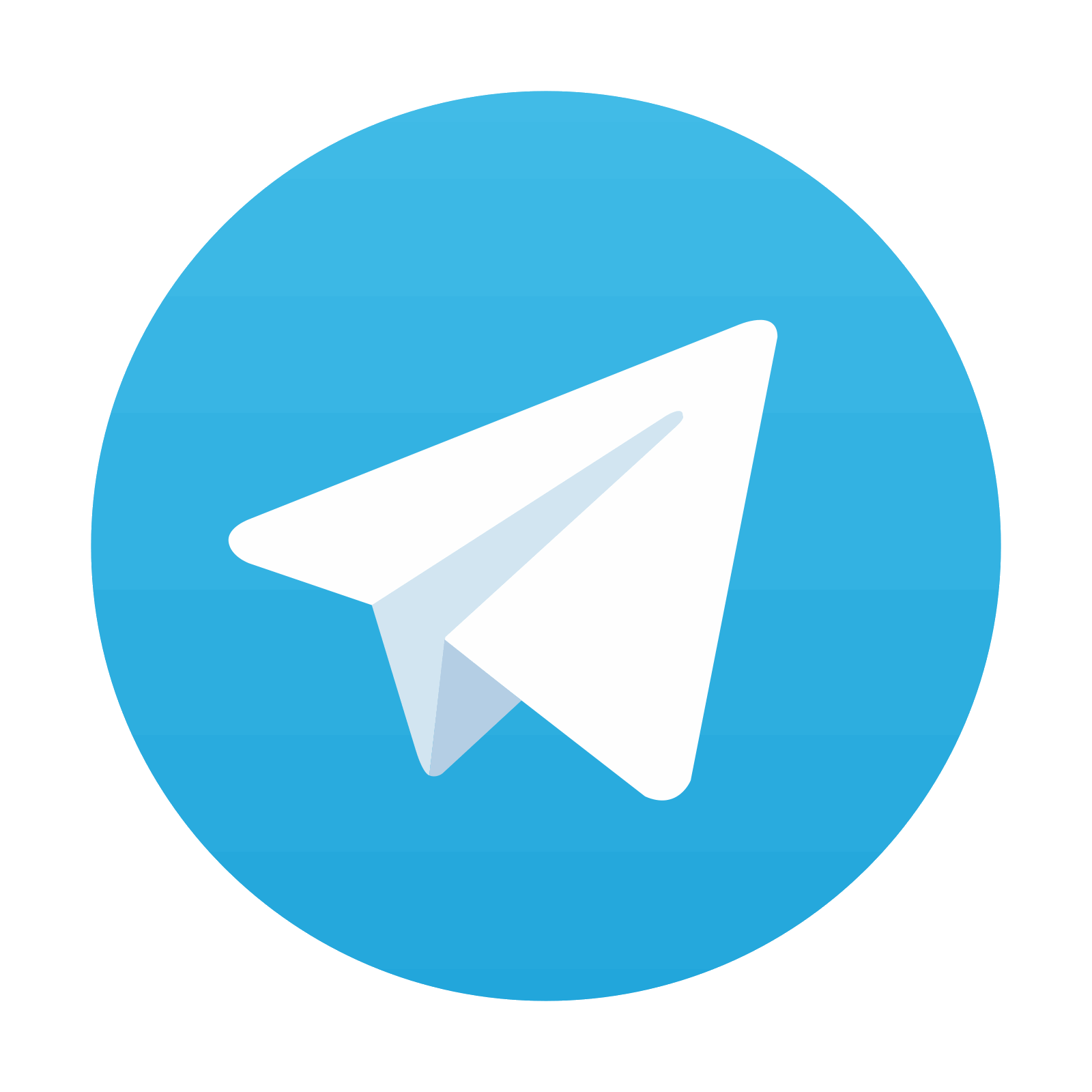
Stay updated, free articles. Join our Telegram channel
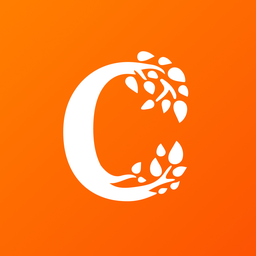
Full access? Get Clinical Tree
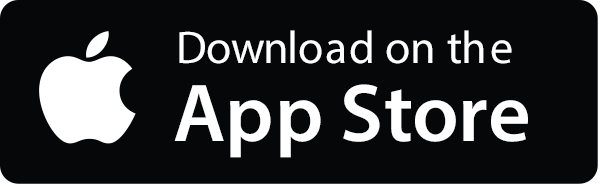
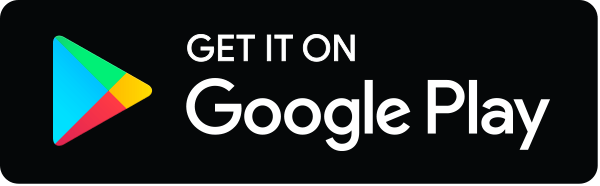