Fig. 11.1
Schematic drawing of a cardiomyocyte with the localisation of the eight most common sarcomeric HCM disease genes in the thick and thin filament. Additionally, the localisation non-contractile proteins implicated in HCM is indicated
CSRP3 codes for muscle LIM protein (MLP), a protein specific for cardiac and slow skeletal muscle. It was initially described as a Z-disc component (Knoll et al. 2002), but later found to be primarily cytoplasmic (Geier et al. 2008), with potential functions also in the nucleus (Boateng et al. 2009). Four different missense mutations in CSRP3 were found to be causative for HCM, and the second zinc finger of the first LIM domain appears to be a mutational hotspot. The best studied mutation is CSRP3 p. C58G. Linkage analysis and co-segregation in a large German family underline the pathogenicity of the mutation, and functional studies suggest that the mutation renders the protein less stable; hence, a lack on functional MLP protein could be the driver of HCM in this case (Gehmlich et al. 2004; Geier et al. 2008). Mutations in CSRP3 are rare and account for less than 1 % of the HCM cases.
CSRP3 p. W4R was initially described as a DCM-causing mutation (Knoll et al. 2002) and later also found in HCM patients (Bos et al. 2006). However, it is now known as a common variant (with an allelic frequency of approx. 0.9 % in the German population (Geier et al. 2008), 0.37 % at Exome Variant Server, EVS, http://evs.gs.washington.edu/EVS/) and discussed as a modifier gene for HCM (Knoll et al. 2010).
For the other non-contractile HCM genes, the majority of mutations were found by candidate gene screening approaches, and an attribution of the pathogenic role was based on the presence of a mutation in single individuals with HCM and its absence in small control cohorts (a few hundred individuals). Most reports fail to show cosegregation in large families and/or genome-wide linkage, which is the gold standard to describe novel disease genes. With more sequencing data being available now due to next-generation sequencing (NGS) technology, some reported HCM-causing mutations have now been found at low frequencies in the normal population (Andreasen et al. 2013). Future studies will reveal how these variants contribute to HCM, e.g. as modifier genes. So far MURFs and FHL1 have been identified as modifier genes in HCM (Christodoulou et al. 2014; Su et al. 2014).
TTN mutations have been found in HCM patients, with a high proportion being missense variants (Lopes et al. 2013b). However, variants in TTN seem to occur also in the normal population and future research is needed to classify and evaluate them (see below).
Overall, mutations in genes coding for non-contractile proteins are rare and certainly do not account for the ‘missing 40 %’ of HCM patients, where no mutation in the common HCM genes has been identified. Indeed, the majority of those 40 % of HCM patients are unlikely to have Mendelian disease. Also they behave clinically as a distinct disease entity, e.g. they have milder microvascular dysfunction and less fibrosis (Olivotto et al. 2011).
11.3.2.3 Phenocopies of HCM and Mitochondrial Disease
The main phenocopies of HCM are Wolff-Parkinson-White syndrome caused by mutations in PRKAG2 [the gamma2 subunit of AMP kinase (Murphy et al. 2005)], Fabry disease caused by mutations in GLA [alpha-galactosidase (Monserrat et al. 2007a)] and Danon disease caused by mutations in LAMP2 [lysosome-associated membrane protein 2 (Yang et al. 2005)]. Of note, Fabry disease and Danon disease are X-linked. Likewise, mutations in FHL1 (coding for four-and-a-half-LIM-domains 1) cause X-linked myopathy with HCM, but isolated HCM cases have been reported (Friedrich et al. 2012; Hartmannova et al. 2013).
11.3.3 Pathomechanisms of HCM
Following closely behind the genetics of HCM, in vitro and animal model experiments have yielded significant insights into disease mechanisms. On a cellular level, HCM is associated with abnormal sarcomeric calcium handing, energy homeostasis, biomechanical stress signalling, fibrosis and microvascular changes, which are all proposed to underlie or contribute to the pathogenesis of disease.
The vast majority of HCM mutations affect proteins of the cardiac contractile apparatus. HCM mutations typically increase calcium sensitivity and actin-dependent ATPase activity (Robinson et al. 2002), leading to increased myofilament contractility (Robinson et al. 2007; Sequeira et al. 2013; Witt et al. 2001). Increased cross-bridge cycling and ATPase activity (Belus et al. 2008) has a higher energy requirement to produce a given force—‘tension cost’ (Frey et al. 2006). This points at inefficient energy usage and relative energy depletion, which itself is proposed to drive disease pathogenesis (Ashrafian et al. 2003).
The energy depletion hypothesis is supported by the finding that HCM patients have a lower ratio of cardiac phosphocreatine (PCr) to adenosine triphosphate (ATP), independent of the underlying HCM mutation and occurring prior to the development of hypertrophy (Crilley et al. 2003). Cellular energy depletion has also been observed in mouse models and in vitro experiments (Ferrantini et al. 2009; Song et al. 2013; Witjas-Paalberends et al. 2014). Additionally, an HCM-like phenotype develops in a range of metabolic and mitochondrial disorders which compromise cellular energetics. Treatment of HCM patients with perhexiline, which optimises cardiac substrate metabolism with a shift towards carbohydrate use, improves the PCr/ATP ratio and improves functional capacity (Abozguia et al. 2010).
Downstream of the sarcomere, HCM mutations are associated with altered calcium signalling and calcium handling proteins such as SERCA2a and phospholamban (PLN). Changes in calcium kinetics have been proposed to underlie arrhythmogenesis, which can occur in the absence of significant hypertrophy (Knollmann et al. 2003). Cardiac troponin T HCM mouse models show alterations in SERCA2a:PLN ratio, PLN phosphorylation pattern and sarcoplasmic reticulum calcium uptake (Guinto et al. 2009). In support of a disease-causing role for aberrant calcium signalling, neonatal adenoviral delivery of SERCA2a to a tropomyosin mutant HCM mouse model was found to delay the development of hypertrophy (Pena et al. 2010).
Patients with HCM develop significant cardiac fibrosis, although the molecular triggers remain incompletely defined (Ho et al. 2010). Fibrosis occurs early, often prior to overt hypertrophy, and is a cause of arrhythmia and diastolic dysfunction and an important predictor of adverse survival (O’Hanlon et al. 2010). In addition, intimal hyperplasia and medial hypertrophy of the intramyocardial microvasculature lead to reduced flow and ischaemia, further promoting fibrosis and leading to impaired contractile function (Olivotto et al. 2011).
The pathomechanisms of HCM caused by mutations in non-contractile proteins remain enigmatic and will be the subject of future research. A role for downstream biomechanical stress signalling is suggested by identification of mutations in signalling molecules such as CSRP3 and ANKRD1. Dissecting the cellular pathomechanisms in HCM holds great promise for developing clinical treatments, for patients with not only cardiomyopathy but also other diseases characterised by inappropriate hypertrophy or heart failure.
11.4 Next-Generation Sequencing: ‘Opening Pandora’s Box’?
Next-generation sequencing (NGS) technology has dramatically enhanced understanding of the genetic architecture of disease. For genetic cardiomyopathies, NGS holds great promise for elucidating the more complex genetic background to these diseases, for example, in HCM patients where a mutation is not identified by traditional candidate gene screening. However, the increased sensitivity of genetic sequencing also raises the substantial challenge of how to interpret variants of unknown clinical significance (VUS) and whether they are causal, contributory or bystanders to a disease phenotype.
Rare coding variation in the genome has historically been significantly underestimated (Tennessen et al. 2012). Overall, in a study of over 5,000 exomes, over half the sequence variants identified were unique to one patient (Pan et al. 2012), although this was substantially lower for known cardiomyopathy genes. Previously, pathogenicity has been ascribed to ‘mutations’ identified in candidate gene sequencing studies on the basis of absence from a small sample of controls. It is now clear that many of these variants are present in the normal population at low frequency, casting significant doubt on their pathogenicity in disease (Andreasen et al. 2013; Christensen et al. 2010).
NGS thus poses a significant challenge to researchers—how to pick out genuine disease-causing mutations from rare, benign variants existing at low frequency in the genome. Furthermore, genetic variants may not be acting in isolation, but as compound mutations, which are disease-causing when in the presence of another mutation, or as modifiers of the phenotype. Early use of targeted high-throughput sequencing in patients with HCM hints at the level of genetic complexity to be unravelled: 11 % had multiple candidate variants within the eight sarcomeric genes alone. Over 40 % of this HCM clinical cohort also had VUS in ion channel and AC candidate genes (Lopes et al. 2013b).
NGS has also been employed to overcome the technical challenges inherent in sequencing the enormous TTN gene, containing 363 exons (Herman et al. 2012). Using massively parallel sequencing, truncating variants were identified in 22 % patients with idiopathic DCM, significantly higher than in HCM or controls. Confirming pathogenicity in a given patient remains difficult, however, given that TTN truncating variants were also found to exist in the control population at a frequency of 3 %. Presumably pathogenicity is influenced by as yet unknown modifying genetic or epigenetic loci, alongside environmental factors such as hypertension.
The strongest evidence for pathogenicity for a given mutation comes from linkage analysis or co-segregation with a phenotype—as established for the sarcomeric HCM genes—but frequently this is not possible, for example, in small families, for sporadic disease or where penetrance is low. In general, disease-causing variants would be expected to have a low frequency in libraries of control populations (e.g. 1,000 Genomes Project, the Exome Variant Server http://evs.gs.washington.edu/EVS/ and Exome Aggregation Consortium Browser http://exac.broadinstitute.org/) and to be enriched in disease cases (e.g. ClinVar http://www.ncbi.nlm.nih.gov/clinvar/). Pathogenic mutations are more likely to be those which disrupt the amino acid reading frame. In silico or isolated in vitro effects on protein function or structure are supportive but are a ‘low bar’ to meet (Watkins 2013) and have low sensitivity and specificity. Recapitulation of the phenotype in animal models, with subsequent rescue by silencing the variant, is strong evidence but costly and time-consuming. Hence, new cost-effective and efficient test systems, e.g. cardiac-specific cellular assays, are required to evaluate the pathogenicity of variants.
Overall, the resources needed for NGS interpretation are substantial and for the moment remain prohibitive outside a research environment. In addition to VUS assessment and interpretation, there are challenges inherent in bioinformatic processing, management of incidental findings and updating results over time as VUS are identified as benign or pathogenic (Dewey et al. 2014). As large databanks of genomes are acquired and automation and filtering of sequence results are improved, NGS will become an extremely powerful tool in probing beyond the ‘one mutation, one gene’ description of genetic disease of the heart.
Acknowledgement
KG is supported by a British Heart Foundation Grant (FS/12/40/29712).
References
Abozguia K, Elliott P, McKenna W, Phan TT, Nallur-Shivu G, Ahmed I, Maher AR, Kaur K, Taylor J, Henning A, Ashrafian H, Watkins H, Frenneaux M (2010) Metabolic modulator perhexiline corrects energy deficiency and improves exercise capacity in symptomatic hypertrophic cardiomyopathy. Circulation 122(16):1562–1569. doi:10.1161/CIRCULATIONAHA.109.934059 CrossRefPubMed
Ackermann MA, Kontrogianni-Konstantopoulos A (2011) Myosin binding protein-C: a regulator of actomyosin interaction in striated muscle. J Biomed Biotechnol 2011:636403. doi:10.1155/2011/636403 CrossRefPubMedCentralPubMed
Afonso LC, Bernal J, Bax JJ, Abraham TP (2008) Echocardiography in hypertrophic cardiomyopathy: the role of conventional and emerging technologies. JACC Cardiovasc Imaging 1(6):787–800. doi:10.1016/j.jcmg.2008.09.002 CrossRefPubMed
Alders M, Jongbloed R, Deelen W, van den Wijngaard A, Doevendans P, Ten Cate F, Regitz-Zagrosek V, Vosberg HP, van Langen I, Wilde A, Dooijes D, Mannens M (2003) The 2373insG mutation in the MYBPC3 gene is a founder mutation, which accounts for nearly one-fourth of the HCM cases in the Netherlands. Eur Heart J 24(20):1848–1853CrossRefPubMed
Andreasen C, Nielsen JB, Refsgaard L, Holst AG, Christensen AH, Andreasen L, Sajadieh A, Haunso S, Svendsen JH, Olesen MS (2013) New population-based exome data are questioning the pathogenicity of previously cardiomyopathy-associated genetic variants. Eur J Hum Genet 21(9):918–928. doi:10.1038/ejhg.2012.283 CrossRefPubMedCentralPubMed
Arad M, Penas-Lado M, Monserrat L, Maron BJ, Sherrid M, Ho CY, Barr S, Karim A, Olson TM, Kamisago M, Seidman JG, Seidman CE (2005) Gene mutations in apical hypertrophic cardiomyopathy. Circulation 112(18):2805–2811. doi:10.1161/CIRCULATIONAHA.105.547448 CrossRefPubMed
Arbustini E, Narula N, Tavazzi L, Serio A, Grasso M, Favalli V, Bellazzi R, Tajik JA, Bonow RD, Fuster V, Narula J (2014) The MOGE(S) classification of cardiomyopathy for clinicians. J Am Coll Cardiol 64(3):304–318. doi:10.1016/j.jacc.2014.05.027 CrossRefPubMed
Arimura T, Bos JM, Sato A, Kubo T, Okamoto H, Nishi H, Harada H, Koga Y, Moulik M, Doi YL, Towbin JA, Ackerman MJ, Kimura A (2009) Cardiac ankyrin repeat protein gene (ANKRD1) mutations in hypertrophic cardiomyopathy. J Am Coll Cardiol 54(4):334–342. doi:10.1016/j.jacc.2008.12.082 CrossRefPubMed
Ashrafian H, Redwood C, Blair E, Watkins H (2003) Hypertrophic cardiomyopathy: a paradigm for myocardial energy depletion. Trends Genetic 19(5):263–268. doi:10.1016/S0168-9525(03)00081-7 CrossRef
Azibani F, Muchir A, Vignier N, Bonne G, Bertrand AT (2014) Striated muscle laminopathies. Semin Cell Dev Biol 29C:107–115. doi:10.1016/j.semcdb.2014.01.001 CrossRef
Becker E, Navarro-Lopez F, Francino A, Brenner B, Kraft T (2007) Quantification of mutant versus wild-type myosin in human muscle biopsies using nano-LC/ESI-MS. Anal Chem 79(24):9531–9538. doi:10.1021/ac701711h CrossRefPubMed
Belus A, Piroddi N, Scellini B, Tesi C, D’Amati G, Girolami F, Yacoub M, Cecchi F, Olivotto I, Poggesi C (2008) The familial hypertrophic cardiomyopathy-associated myosin mutation R403Q accelerates tension generation and relaxation of human cardiac myofibrils. J Physiol 586(Pt 15):3639–3644. doi:10.1113/jphysiol.2008.155952 CrossRefPubMedCentralPubMed
Boateng SY, Senyo SE, Qi L, Goldspink PH, Russell B (2009) Myocyte remodeling in response to hypertrophic stimuli requires nucleocytoplasmic shuttling of muscle LIM protein. J Mol Cell Cardiol 47(4):426–435. doi:10.1016/j.yjmcc.2009.04.006 CrossRefPubMedCentralPubMed
Bos JM, Poley RN, Ny M, Tester DJ, Xu X, Vatta M, Towbin JA, Gersh BJ, Ommen SR, Ackerman MJ (2006) Genotype-phenotype relationships involving hypertrophic cardiomyopathy-associated mutations in titin, muscle LIM protein, and telethonin. Mol Genet Metab 88(1):78–85. doi:10.1016/j.ymgme.2005.10.008 CrossRefPubMedCentralPubMed
Cahill TJ, Ashrafian H, Watkins H (2013) Genetic cardiomyopathies causing heart failure. Circ Res 113(6):660–675. doi:10.1161/CIRCRESAHA.113.300282 CrossRefPubMed
Carballo S, Robinson P, Otway R, Fatkin D, Jongbloed JD, de Jonge N, Blair E, van Tintelen JP, Redwood C, Watkins H (2009) Identification and functional characterization of cardiac troponin I as a novel disease gene in autosomal dominant dilated cardiomyopathy. Circ Res 105(4):375–382. doi:10.1161/CIRCRESAHA.109.196055 CrossRefPubMed
Carrier L, Schlossarek S, Willis MS, Eschenhagen T (2010) The ubiquitin-proteasome system and nonsense-mediated mRNA decay in hypertrophic cardiomyopathy. Cardiovasc Res 85(2):330–338. doi:10.1093/cvr/cvp247 CrossRefPubMedCentralPubMed
Chauveau C, Rowell J, Ferreiro A (2014) A rising titan: TTN review and mutation update. Hum Mutat 35(9):1046–1059. doi:10.1002/humu.22611 CrossRefPubMed
Christensen AH, Benn M, Tybjaerg-Hansen A, Haunso S, Svendsen JH (2010) Missense variants in plakophilin-2 in arrhythmogenic right ventricular cardiomyopathy patients–disease-causing or innocent bystanders? Cardiology 115(2):148–154. doi:10.1159/000263456 CrossRefPubMed
Christiaans I, Nannenberg EA, Dooijes D, Jongbloed RJ, Michels M, Postema PG, Majoor-Krakauer D, van den Wijngaard A, Mannens MM, van Tintelen JP, van Langen IM, Wilde AA (2010) Founder mutations in hypertrophic cardiomyopathy patients in the Netherlands. Neth Heart J 18(5):248–254CrossRefPubMedCentralPubMed
< div class='tao-gold-member'>
Only gold members can continue reading. Log In or Register a > to continue
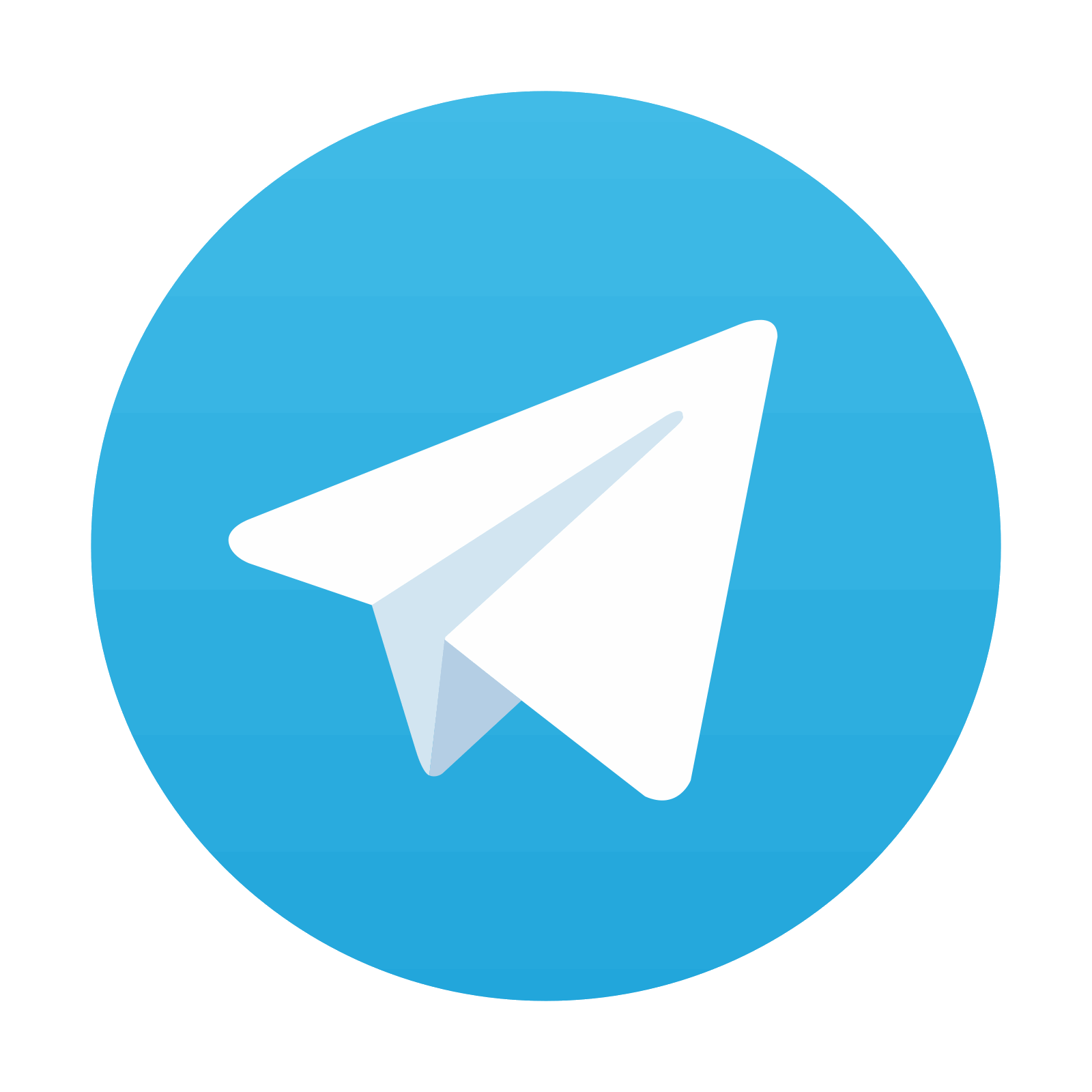
Stay updated, free articles. Join our Telegram channel
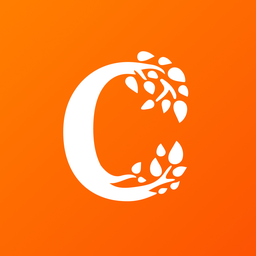
Full access? Get Clinical Tree
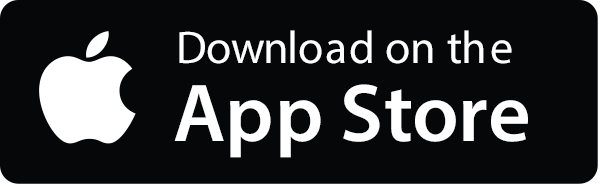
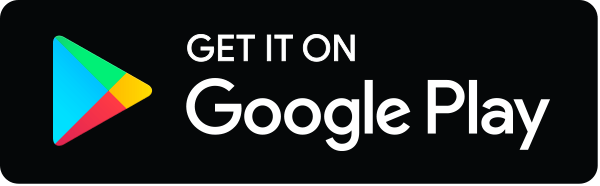