Background
Speckle-tracking echocardiography (STE) can be used to quantify wall strain in 3 dimensions and thus has the potential to improve the identification of hypokinetic but viable myocardium on dobutamine stress echocardiography (DSE). However, if different myocardial layers respond heterogeneously, STE-DSE will have to be standardized according to strain dimension and the positioning of the region of interest. Therefore, the aim of this study was to create a high-resolution model for ejection time (ET) strain and tissue flow in 4 myocardial layers at rest, during hypoperfusion, and during dobutamine challenge to assess the ability of STE-DSE to detect deformation and functional improvement in various layers of the myocardium.
Methods
In 10 open chest pigs, the left anterior descending coronary artery was constricted to a constant stenosis, resulting in 35% initial flow reduction. Fluorescent microspheres were used to measure tissue flow. High-resolution echocardiography was performed epicardially to calculate ET strain in 4 myocardial layers in the radial, longitudinal, and circumferential directions using speckle-tracking software. Images were obtained at rest, during left anterior descending coronary artery constriction (hypoperfusion), and during a subsequent dobutamine stress period.
Results
Dobutamine stress at constant coronary stenosis increased flow in all layers. ET strain increased predominantly in the midmyocardial layers in the longitudinal and circumferential directions, whereas subendocardial strain did not improve in either direction.
Conclusion
Dobutamine stress influences ET strain differently in the various axes and layers of the myocardium and only partially in correspondence to tissue flow. Longitudinal and circumferential functional reserve opens the potential for the specific detection of midsubendocardial viable tissue by high-resolution STE.
Speckle tracking echocardiography (STE) uses spontaneous echocardiographic acoustic markers and quantifies regional myocardial deformation and its time course (strain and strain rate) with high temporal resolution. STE also has high spatial resolution and allows radial, longitudinal, and circumferential strain analyses in all segments of the left ventricle. As such, speckle tracking could potentially be used as a bedside tool to improve the assessment of the regional functional status of the myocardium. By definition, this technique will be influenced by the described heterogeneity in laminar extension, shear, and thickening. Additionally, assessment of the potential viability of hypocontracting myocardium through the infusion of contractility-enhancing drugs (ie, dobutamine stress echocardiography [DSE]) can also be influenced by this heterogeneous response in different myocardial layers.
We therefore aimed to investigate myocardial strain during ejection time (ET), using high-resolution epicardial STE to measure regional deformation in 4 different layers of the left ventricle with varying perfusion and a concomitant dobutamine challenge. If regional and transmural layer heterogeneity of deformation do occur, the region of interest (ROI) and segmental assessment can possibly be optimized on the basis of detailed knowledge of this heterogeneous response in various pathophysiologic settings. Additionally, if contractile reserve can be demonstrated in the subendocardial and midendocardial to subendocardial layers, high-resolution DSE using STE might potentially be used as a future method to define such regional viability and predict subendocardial improvement of function after revascularization. In the present study, we characterized such a response using a well-defined animal model of perfusion-contraction matching in acute ischemic (anterior wall) and adjacent tissue (lateral wall).
Materials and Methods
Animals
The investigation was conducted in accordance with the Guide for the Care and Use of Laboratory Animals published by the National Institutes of Health (Publication No 85-23, revised 1996), and the experimental protocol was approved by the local steering committee of the Norwegian Experimental Animal Board. Ten domestic pigs (cross-breeds of Norwegian Landrace and Yorkshire) from both sexes weighing 37.7 ± 2.4 kg were fasted overnight before the experiment, with free access to water.
Instrumentation
Intramuscular ketamine (20 mg/kg; Pfizer AS, Oslo, Norway), midazolam (1 mg/kg; B. Braun Melsungen AG, Melsungen, Germany), and atropine (1 mg, Nycomed Pharma AS, Oslo, Norway) were used as premedication. Anesthesia was induced with intravenous boluses of pentobarbital sodium (10 mg/kg; Abbott Scandinavia AB, Solna, Sweden) and fentanyl (0.01 mg/kg; Hameln Pharmaceuticals GmbH, Hameln, Germany), followed by continuous infusions of pentobarbital sodium (4 mg/kg/h) and fentanyl (0.02 mg/kg/h). The pigs were tracheotomized and intubated, and ventilation was maintained with an air-oxygen mixture (FiO 2 = 0.5) using a volume-controlled respirator. The initial tidal volume of 100 ml/kg and a ventilation frequency of 13 to 15 breaths/min were adjusted according to arterial blood gas samples (P co 2 = 4.0-5.0 kPa). The depth of anesthesia, blood pressure, electrocardiogram, respiration, and temperature were monitored continuously. Intravenous boluses of heparin (2500 IU; LEO Pharma A/S, Ballerup, Danmark) and amiodarone (300 mg; Sanofi-Synthélabo, Stockholm, Sweden) were given to prevent blood clotting of catheters and cardiac arrhythmias. Central venous pressure was monitored through the right jugular vein and held constant at 4 ± 1 mm Hg by infusion of 0.9% NaCl enriched with glucose (1.25 g/L). For systolic and diastolic blood pressure and mean arterial pressure recordings and arterial blood sampling in the descending thoracic aorta, a catheter inserted through the left femoral artery was used. A sternotomy was performed, and the pericardium was opened at the base, while maintaining apical fixation by leaving the apical pericardium intact helped reduce motion artifacts. Transit time flow probes (CardioMed CM-4000; Medi-Stim AS, Horten, Norway) were placed on the pulmonary artery, the left anterior descending coronary artery (LAD), and the circumflex coronary artery. Proximal to the LAD flow probe, an elastic rubber band was looped around the LAD for flow reduction. At the end of the experiment, the animals were euthanized by an overdose of KCl and pentobarbital sodium. The left ventricles were then harvested for later analysis of regional flow distribution.
Echocardiography
Echocardiographic high-resolution B-mode images were acquired using a 5-MHz to 12-MHz epicardial probe and a Philips iE33 ultrasound scanner (Philips Medical Systems, Andover, MA) with which an average pixel size of 0.054 mm could be achieved. To avoid near-field and motion artifacts, a 1-cm-thick jelly pad and a layer of fluid jelly were fixed using a plastic bag taped to the probe. Long-axis and short-axis two-dimensional images of the midanterior and midlateral wall were acquired at a frame rate of 100 to 115 Hz. High frame rates were achieved without a reduction in spatial resolution by using an image-sector angle of 30° and maximal zooming. Two-dimensional images of the left ventricular cavity served as orientation to ensure repeated scans of the same regions. Aortic flow by Doppler measurements was acquired for the timing of aortic valve opening and closure to calculate strain in the ET period at all stages of the protocol. The two-dimensional high-resolution acquisitions were analyzed using offline dedicated software (Syngo Velocity Vector Imaging; Siemens Medical Systems, Erlangen, Germany). For radial strain, 80 points were placed at end-diastole in the long-axus and short-axis acquisitions of the myocardial wall, 32 points for each myocardial layer, as shown in Figure 1 . For longitudinal and circumferential strain, 64 points, 16 in each layer, were positioned in long-axis and short-axis end-diastolic frames, respectively ( Figure 1 ). Coordinates for each point and frame for the time course of 3 cardiac cycles were calculated. Customized software from our laboratory transformed the coordinates into a new coordinate system aligning axes into radial, circumferential, and longitudinal directions. Strain was calculated from either coordinate of the corresponding radial, longitudinal, or circumferential axis. In the radial direction, the difference of mean values of two parallel rows of points served to calculate Lagrangian strain ( Figure 1 ). The final radial strain values were calculated as the average from both long-axis and short-axis projections. For longitudinal and circumferential strain, 4 points of 4 vertical rows consisting of 16 points represented one myocardial layer. Two strains between the midpoint coordinate of the first and third and the second and fourth row were calculated, and both values were averaged. Transmural strain in all directions was calculated as the average of all 4 layer strains. Before selecting curves for statistical analyses, a subjective quality assessment was performed. For each curve obtained, the following criteria had to be fulfilled: (1) points in the epicardial and endocardial borders were visually observed to follow these structures, (2) the motion of the majority (>80%) of points had to follow a constant track and not cross the track of neighboring points, (3) points had to not interfere with the image border, and (4) B-mode images had to be free of artifacts. Acquisitions not fulfilling these criteria were discarded.

Fluorescent Regional Myocardial Flow
Tissue flow measurements were performed with 5 different color microspheres following a standard protocol, which was carried out by injection of the fluorescent microspheres at the end of the experimental stages. The 15-μm spheres (Dye-Trak V+R; Triton Technology, San Diego, CA) were injected within 30 seconds through a catheter in the left atrium at a concentration of 5 × 10 6 /10 mL saline at rest, under LAD constriction and during the infusion of 0.25, 5, and 10 μg/kg/min dobutamine. Starting with the injection of microspheres, a reference flow sample from the femoral artery was taken with a total volume of 8.28 mL at a flow velocity of 4.12 mL/min. At the end of the experiments, the heart was excised, and the midanterior and midlateral walls were divided into 4 myocardial layers: the subepicardium, midsubepicardium, midsubendocardium, and subendocardium. Pieces of approximately 2 g were analyzed, and the tissue blood flow rate was calculated in milliliters per minute per gram, according to standard procedures.
Experimental Protocol
Complete data sets were obtained at 7 time points during the experiment, including hemodynamic recordings, epicardial echocardiography, and color microsphere–based myocardial blood flow assessments. Baseline recordings were obtained 30 minutes after completed instrumentation. Thereafter, the coronary flow was reduced by snaring the LAD a few millimeters proximal to the flow probe with an elastic ligation. Because of the hyperemic response, a time window of 30 minutes was required to obtain a steady-state 30% to 50% flow reduction. Finally, dobutamine was added in stepwise increasing doses of 0.1, 0.25, 2.5, 5, and 10 μg/kg/min, and recordings (flow reduction plus dobutamine) were obtained immediately after a new steady state was reached for each dose (∼3 minutes). For dobutamine maximum and flow reduction, the dobutamine doses with the highest transmural vector ET strain (sum of all strains) in the anterior, hypoperfused wall were selected in each animal for further flow and strain analyses. The same dobutamine dose of each animal was also used for ET strain calculation in the lateral wall and tissue flow analyses.
Statistical Analyses
For statistical analyses, we calculated the mean values during ET for strain because these data were more reproducible than peak values. Differences of resting condition, flow reduction, and dobutamine maximum and flow reduction for tissue flow and ET strain in all directions and layers were calculated by analysis of variance for repeated measurements, with simple contrasts. The same procedure was also used to express differences between the subendocardial and each of the other 3 layers and in hemodynamic parameters to compare resting values with the other experimental stages. For comparison of ET strain and flow at dobutamine maximum and flow reduction and dobutamine 10 μg/kg/min and flow reduction, a paired t test was performed. The correlations between tissue flow and ET strain at the 3 different experimental conditions from both walls were tested by multiple nonlinear regressions. Best fit of curves was tested by fractional polynomials with two members. The results are expressed as the coefficient of determination ( r 2 ) and P value. In all statistical analyses, a P value ≤ .05 was considered to indicate statistical significance. Unless stated otherwise, all data are expressed as mean ± SEM.
Results
Effect of Flow Reduction and Dobutamine on General Hemodynamics
Table 1 shows the hemodynamic parameters during the experiment. Systolic blood pressure, mean arterial pressure and cardiac output decreased and central venous pressure increased during LAD flow reductions. Cardiac output and heart rate increased continuously with increasing dobutamine doses, whereas both systolic blood pressure and mean arterial pressure were highest at dobutamine 2.5 μg/kg/min. LAD flow at rest, measured by the LAD transit time flow probe, was moderately reduced (35 ± 13%, P = .001) after application of the occluder. This flow reduction did not reduce the flow in the area of the circumflex coronary artery. Importantly, dobutamine application approximately doubled circumflex flow and increased LAD flow to the resting flow level.
Intervention | Systolic BP (mm Hg) | Diastolic BP (mm Hg) | MAP (mm Hg) | CVP (mm Hg) | HR (beats/s) | Cx flow (mL/min) | LAD flow (mL/min) | CO (L/min) | SV (mL/min) |
---|---|---|---|---|---|---|---|---|---|
Resting | 134 ± 6 | 64 ± 3 | 88 ± 4 | 5.9 ± 0.5 | 80 ± 5 | 38 ± 4 | 41 ± 3 | 3.10 ± 0.17 | 39.6 ± 9.0 |
FR | 117 ± 5 ∗ | 60 ± 4 | 78 ± 4 ∗ | 6.9 ± 0.5 ∗ | 88 ± 7 | 45 ± 6 | 27 ± 3 ∗ | 2.80 ± 0.13 ∗ | 32.9 ± 8.6 ∗ |
FR Dob 0.1 | 117 ± 4 ∗ | 58 ± 2 | 77 ± 3 ∗ | 7.4 ± 0.7 ∗ | 88 ± 6 | 42 ± 6 | 27 ± 2 ∗ | 2.69 ± 0.12 ∗ | 31.9 ± 8.1 ∗ |
FR Dob 0.25 | 120 ± 5 ∗ | 61 ± 2 | 81 ± 3 | 6.7 ± 0.6 | 89 ± 6 | 44 ± 5 | 27 ± 3 ∗ | 2.80 ± 0.11 ∗ | 33.2 ± 8.4 ∗ |
FR Dob 2.5 | 142 ± 6 | 67 ± 4 | 92 ± 4 | 6.1 ± 0.6 | 95 ± 5 ∗ | 56 ± 6 ∗ | 32 ± 3 ∗ | 3.51 ± 0.14 | 37.9 ± 9.8 |
FR Dob 5.0 | 134 ± 8 | 58 ± 4 | 84 ± 4 | 5.7 ± 0.5 | 107 ± 5 ∗ | 64 ± 6 ∗ | 34 ± 4 ∗ | 4.23 ± 0.15 ∗ | 40.4 ± 10.0 |
FR Dob 10.0 | 129 ± 10 | 54 ± 6 | 78 ± 5 | 5.8 ± 0.7 | 126 ± 7 ∗ | 76 ± 7 ∗ | 43 ± 5 | 4.85 ± 0.26 ∗ | 40.8 ± 11.1 |
Basal Strain and Flow Gradients
Tissue blood flow, assessed by microspheres and demonstrated in Figure 2 , was not significantly different between layers in the anterior wall at rest, whereas the lateral wall showed significantly higher flow in the subendocardium compared with the subepicardium. ET strain at rest is shown in Figure 3 for each of the 4 layers and in Figure 4 for transmural values. At rest, we found a high transmural gradient in the radial direction in both walls and in the anterior wall in the longitudinal direction, with the highest value in the subendocardium, falling gradually toward the subepicardium. This transmural gradient was observed neither in the circumferential direction of the anterior or lateral wall nor the longitudinal lateral wall.
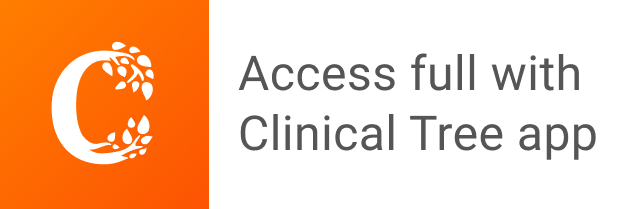