Chapter 17 High altitude and flying



or
Table 17.1 is based on the standard table relating altitude and barometric pressure. However, there are important deviations from the predicted barometric pressure under certain circumstances, particularly at low latitudes.1 At the summit of Everest, the actual barometric pressure was found to be 2.4 kPa (18 mmHg) greater than predicted, and this was crucial to reaching the summit without oxygen. The uppermost curve in Figure 17.1 shows the expected Po2 of air as a function of altitude, while the crosses indicate observed values in the Himalayas that have been consistently higher than expected.
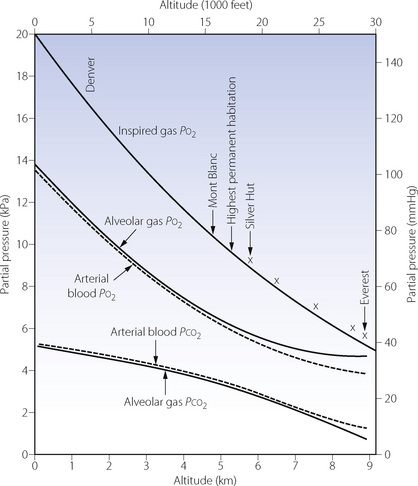
Fig. 17.1 Inspired, alveolar and arterial gas partial pressures at rest, as a function of altitude. The curve for inspired Po2 is taken from standard data in Table 17.1, but the crosses show actual measurements in the Himalayas. The alveolar gas data are from reference 2, and agree remarkably well with the arterial blood data from the simulated ascent of Everest.3
Equivalent Oxygen Concentration
The acute effect of altitude on inspired Po2 may be simulated by reduction of the oxygen concentration of gas inspired at sea level (Table 17.1). This technique is extensively used for studies of hypoxia and for clinical assessment of patients before flying (see below), but there are theoretical reasons why the same inspired Po2 at normal and low barometric pressure may have different physiological effects. These include the density of the gas being breathed and different Pn2 values in the tissues.4
Up to 10 000 m (33 000 ft), it is possible to restore the inspired Po2 to the sea level value by an appropriate increase in the oxygen concentration of the inspired gas (also shown in Table 17.1). Lower inspired Po2 values may be obtained between 10 000 and 19 000 m, above which body fluids boil.
Respiratory System Responses to Altitude1
Acute Exposure to Altitude
Ventilatory changes. At high altitude the decrease in inspired gas Po2 reduces alveolar and therefore arterial Po2. The actual decrease in alveolar Po2 is mitigated by hyperventilation caused by the hypoxic drive to ventilation. However, on acute exposure to altitude, the ventilatory response to hypoxia is very short lived due to a combination of the resultant hypocapnia and hypoxic ventilatory decline (page 73 and Figure 5.6). During the first few days at altitude, this disadvantageous negative feedback is reversed by acclimatisation (see below).
Signs and symptoms. Impairment of night vision is the earliest sign of hypoxia, and may be detected as low as 1200 m (4000 ft). However, the most serious aspect of acute exposure to altitude is impairment of mental performance, culminating in loss of consciousness, which usually occurs on acute exposure to altitudes in excess of 6000 m (about 20 000 ft). The time to loss of consciousness varies with altitude and is of great practical importance to pilots in the event of loss of pressurisation (Figure 17.2). The shortest possible time to loss of consciousness (about 15 seconds) applies above about 16 000 m (52 000 ft) and is governed by lung-to-brain circulation time and the capacity of high energy phosphate stores in the brain (page 363). Impaired mental function as a result of hypoxia is due to both a direct effect of hypoxia on brain tissue and from cerebral vasoconstriction from the resulting hypocapnia.5
Acclimatisation to Altitude
Acclimatisation refers to the processes by which tolerance and performance are improved over a period of hours to weeks after an individual who normally lives at relatively low altitude ascends to a much higher area. Everest has been climbed without oxygen by well-acclimatised lowlanders, although the barometric pressure on the summit would cause rapid loss of consciousness without acclimatisation (Figure 17.2). Adaptation to altitude (described below) refers to physiological differences in permanent residents at high altitude and is quite different from acclimatisation.
Earlier studies of acclimatisation took place in the attractive, though somewhat hostile, environment of high altitude expeditions in many mountain ranges. Technical limitations in these conditions led to two experiments, named Operation Everest II and III, in which volunteers lived in a decompression chamber in which an ascent to the summit of Everest was simulated.6,7 These conditions permitted extensive physiological research to be undertaken at rest and during exercise.
Ventilatory control. Prolonged hypoxia results in several complex changes in ventilation and arterial blood gases, which are shown in Figure 17.3.8 The initial hypoxic drive to ventilation on acute exposure is short lived, and after about 30 minutes ventilation returns to only slightly above normoxic levels with Pco2 just below control levels (Figure 17.3). This poor ventilatory response causes significant arterial hypoxaemia and results in many of the symptoms seen during the first few hours and days at altitude. Over the next few days, ventilation slowly increases with an accompanying reduction of Pco2 and increase in arterial Po2. This increase in Po2 is of relatively small magnitude and can never correct Po2 to normal (sea level) values, but it does seem to be enough to ameliorate most of the symptoms of exposure to acute altitude.
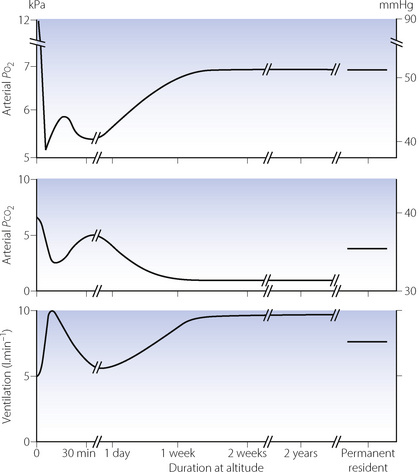
Fig. 17.3 Effects of prolonged hypoxia (equivalent to 4300 m, 14 100 ft) on ventilation and blood gases. The first section of the graph shows the acute hypoxic response and hypoxic ventilatory decline described in Chapter 5. Acclimatisation then takes place, partially restoring Po2 by means of long-term hyperventilation and hypocapnia, a situation that is maintained indefinitely while remaining at altitude. Individuals who reside throughout life at this altitude maintain similar Po2 values with lesser degrees of hyperventilation, but still have a minute ventilation greater than sea level normal.
(After reference 8.)
There are significant differences between species in the rate at which acclimatisation takes place, being just a few hours in most animals, and several days or weeks in humans.4 Both the rate of ascent and the altitude attained influence the speed at which ventilatory acclimatisation occurs, but in humans, most subjects are fully acclimatised within one week.
There are many possible mechanisms to explain the ventilatory changes seen with acclimatisation.8,9 In spite of the low blood Pco2, stimulation of the central chemoreceptors almost certainly plays a part in the hyperventilation that occurs with acclimatisation. It was first suggested, in 1963, that the restoration of cerebrospinal fluid (CSF) pH, by means of bicarbonate transport, might explain this acclimatisation of ventilation to altitude.10 Shortly afterwards Severinghaus and his colleagues measured their own CSF pH during acclimatisation to altitude and showed that it did indeed tend to return towards its initial value of 7.2.10 Subsequent work showed that the time course of changes in CSF pH did not match changes in ventilation,8 with most studies finding a persistent increase in CSF pH during continued exposure to hypoxia.9 Changes in CSF pH therefore seem unlikely to represent an important mechanism of acclimatisation. Other studies, mainly in animals, indicate that acclimatisation represents an increase in the responsiveness of the respiratory centre to hypoxia from both direct effects of prolonged hypoxia on the central nervous system and prolonged maximal afferent input from the peripheral chemoreceptors. This increased responsiveness may be mediated by alterations in the sensitivity to neurotransmitters involved in respiratory control (see Figure 5.4). For example, increased sensitivity to glutamate will directly increase ventilation, or decreasing GABA sensitivity will effectively reduce hypoxic ventilatory decline (page 73).9
In addition to changes affecting the central chemoreceptors, there is evidence that peripheral chemoreceptor sensitivity is increased during prolonged hypoxia, so contributing to the progressive hyperventilation seen with acclimatisation. In humans, the acute hypoxic ventilatory response is increased during the first few days at altitude and for several days after return to sea level. The mechanism of this increased sensitivity to hypoxia is not known, but is independent of changes in Pco2,11 and may reside either with increased sensitivity of the carotid bodies themselves or with the increased responsiveness of the respiratory centre described in the previous paragraph.8,9
Respiratory alkalosis at altitude is counteracted, over the course of a few days, by renal excretion of bicarbonate, resulting in a degree of metabolic acidosis that will tend to increase respiratory drive (see Figure 5.5). This was formerly thought to be the main factor in the ventilatory adaptation to altitude but it now appears to be of minor importance compared to the changes in the central and peripheral chemoreceptors.
Blood gas tensions. Figure 17.3 shows the time course of blood gas changes during acclimatisa-tion and Figure 17.1 shows changes in alveolar gas tensions with altitude in fully acclimatised mountaineers. Alveolar Po2 was found to be unexpectedly well preserved at extreme altitude, and above 8000 m (26 000 ft) tended to remain close to 4.8 kPa (36 mmHg).2 Operations Everest II and III found arterial Po2 values of 3.62 and 4.08 kPa (27 and 31 mmHg) at a pressure equivalent to the summit of Everest (Table 17.2), with an alveolar/arterial Po2 difference of less than 0.3 kPa (2 mmHg) at rest.12 The recent Caudwell Extreme Everest expedition obtained arterial blood samples at 8400 m (27 559 ft) with an average Po2 of 3.28 kPa (24.6 mmHg). There was also a significant alveolar to arterial Po2 difference of 0.72 kPa (5.4 mmHg) which the authors suggested may have resulted from a diffusion barrier to oxygen at such low levels, possibly as a result of sub-clinical pulmonary oedema.13
Table 17.2 Cardiorespiratory data obtained at rest and during exercise at extreme reduction of ambient pressure during the simulated ascent of Everest in a low pressure chamber
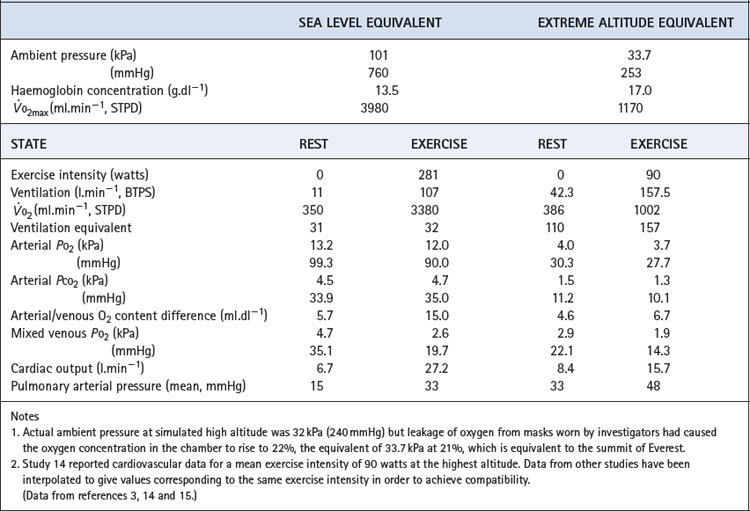
Haemoglobin concentration and oxygen affinity. Anincrease in haemoglobin concentration was the earliest adaptation to altitude to be demonstrated. The recent data from subjects at 8400 m (27 559 ft) reported an increase from 14.8 to 19.3 g.dl−1 which, at the resting value of 54% saturation, maintained an arterial oxygen content of almost 15 ml.dl−1.13 Plasma erythropoietin levels begin to increase within a few hours at altitude, reaching a maximum at 24–48 hours and then declining.16
The haemoglobin dissociation curve at altitude is affected by changes in both pH and 2,3-diphosphoglycerate (DPG) concentration (page 193). 2,3-DPG concentrations increased from 1.7 to 3.8 mmol.l−1 on Operation Everest II.3 It has been estimated that the resultant effect is a leftward shift at extreme altitude, where oxygen loading in the lung takes priority over maintaining Po2 at the point of release.1
Adaptation to Altitude1,17
Adaptation refers to physiological and genetic changes that occur over a period of years to generations by those who have taken up permanent residence at high altitude. There are qualitative as well as quantitative differences between acclimatisation and adaptation but each is remarkably effective. High altitude residents have a remarkable ability to exercise under grossly hypoxic conditions, but their adaptations show many striking differences from those in acclimatised lowlanders. Residents in different high altitude areas of the world have differing adaptations.18
Long-term residence at altitude leads to a reduced ventilatory response to hypoxia, the magnitude of which relates to the product of altitude level and years of residence there.8 This results in a reduction of ventilation compared with an acclimatised lowlander, and a rise in Pco2, though neither of these returns to sea level values (Figure 17.3). High altitude residents maintain similar arterial Po2 values as acclimatised lowlanders in spite of the reduced ventilation and therefore lower alveolar Po2. Pulmonary diffusing capacity must therefore be increased, and depends on anatomical pulmonary adaptations increasing the area available for diffusion by the generation of greater numbers of alveoli and associated capillaries. This adaptation seems not to be inherited, but occurs in children and infants who spend their formative years at altitude. In humans, the development of alveoli by septation of saccules formed in utero occurs mostly after birth (page 250), and it is this process that must be stimulated by hypoxia, though the mechanism of this stimulation remains unknown.19
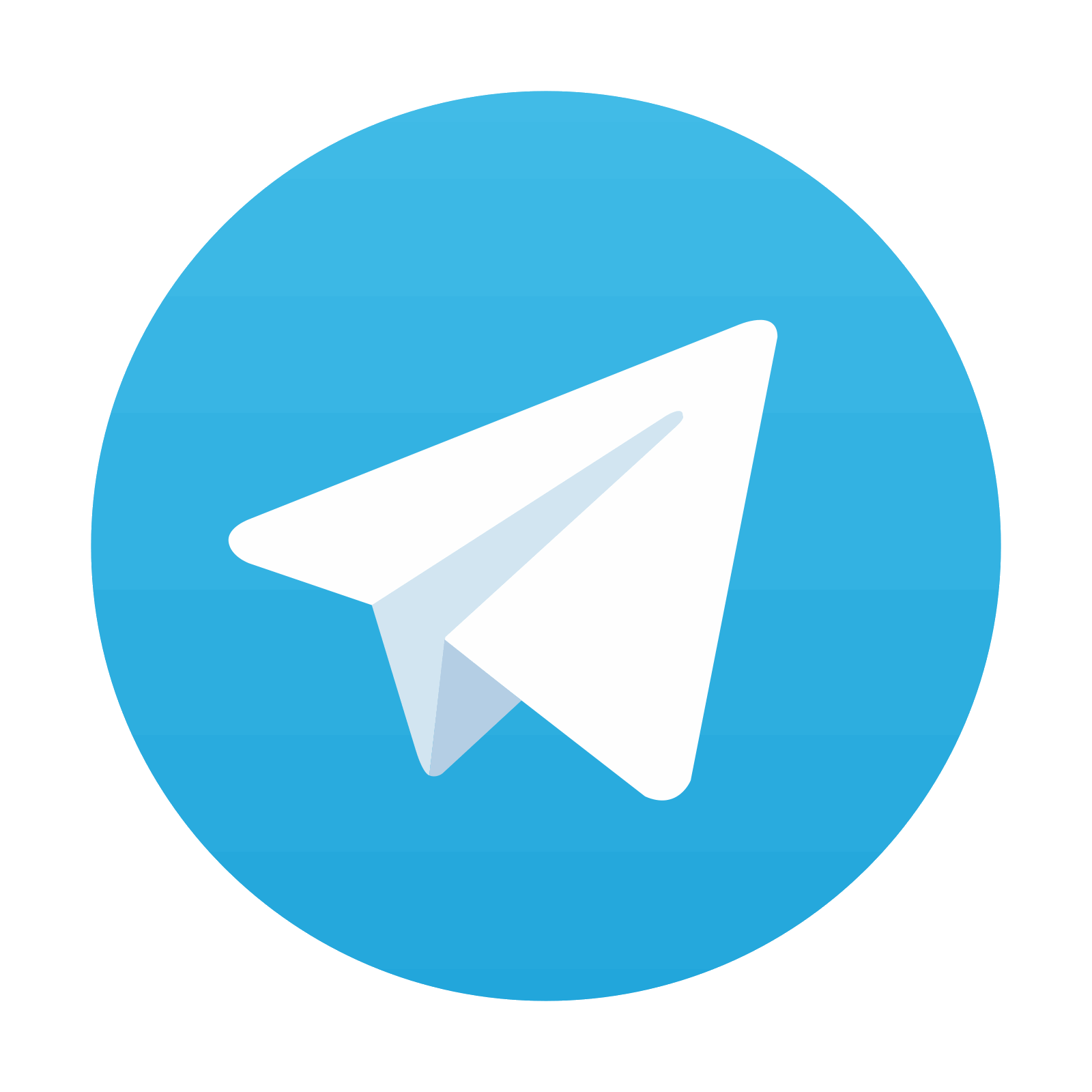
Stay updated, free articles. Join our Telegram channel
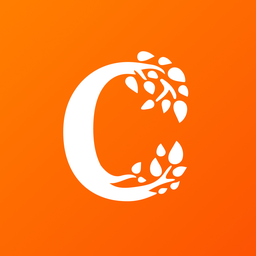
Full access? Get Clinical Tree
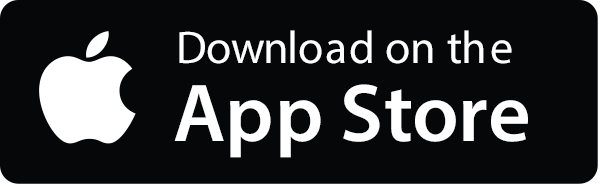
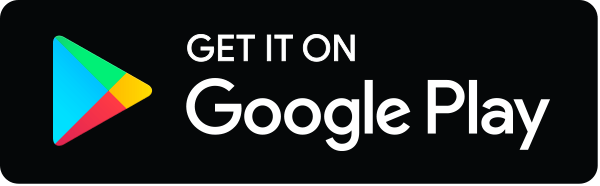