Outline
Technical Issues, 467
Hemodynamic Waveforms, 469
Hemodynamics of Heart Failure With Reduced Ejection Fraction, 474
Hemodynamics for Assessment and Management, 475
Hemodynamics and Advanced Heart Failure, 477
The Hemodynamics of Heart Failure With Preserved Ejection Fraction, 481
Hemodynamic Challenges: Exercise and Volume Loading, 482
The first right heart catheterization (RHC) in humans was performed in 1929 by Dr. Werner Forssmann (on himself), who ultimately shared in the 1956 Nobel Prize in Medicine with Andre Cournard and Dickinson Richards for their work in cardiac catheterization. RHC was further refined by the work of Drs. Bradley and Fife, and in 1969 Drs. Scheinman, Abbot, and Rapaport reported their use of a flow-directed right heart catheter. Following their report of a bedside balloon flotation catheter by Drs. Swan and Ganz in the New England Journal of Medicine in 1970, RHC became widespread both in the catheterization laboratory and in intensive care units for critically ill and unstable patients. Despite advances in noninvasive hemodynamic assessment with echocardiography and cardiac magnetic resonance imaging (MRI), RHC and left heart catheterization (LHC) remains the gold standard for hemodynamic assessment in heart disease. Today, the three predominant reasons to obtain invasive hemodynamics in heart failure include:
- 1.
To resolve diagnostic uncertainty in patients with cryptic symptoms.
- 2.
To assess the suitability for advanced therapies in chronic heart failure.
- 3.
To investigate pulmonary hypertension (PH).
The Society for Cardiovascular Angiography and Interventions and the Heart Failure Society of America Clinical Expert Consensus Document on the Use of Invasive Hemodynamics for the Diagnosis and Management of Cardiovascular Disease summarize the recommendations for invasive hemodynamics for specific clinical scenarios in Table 34.1 .
Hypertrophic Cardiomyopathy |
|
|
|
Valvular heart disease |
|
|
|
Ventricular Function |
|
|
Pericardial Disease |
|
|
|
Pulmonary Hypertension and the Right Ventricle |
|
|
|
|
|
Congenital Heart Disease |
|
|
|
Cardiogenic shock and circulatory support devices |
|
|
|
|
Technical Issues
It is important to recognize that invasive hemodynamics are traditionally measured at rest, in the sedated state, and in a supine position. These limitations should be appreciated when invasive hemodynamics are used to reconcile cryptic symptoms, assess prognosis, or prepare patients for advanced therapies. However, most of our contemporary understanding of heart failure hemodynamics is derived from these resting, supine, and sedated studies. Traditional and novel hemodynamic formulas are illustrated in Fig. 34.1 . Exercise and upright hemodynamics can be obtained in a properly prepared laboratory and are often necessary in certain clinical situations, but are not commonly performed on a routine basis in most clinical catheterization laboratories. The hemodynamic response to various physiologic and/or pharmacologic challenges should also be considered at the time of RHC to maximize the information obtained during this invasive procedure. Finally, it should be appreciated that traditionally obtained hemodynamic measurements lack concomitant measurements of cardiac and/or intravascular volume, so pressure is used as a surrogate for volume.

Pressure measurements in cardiac catheterization are recorded using fluid-filled catheters connected to strain-gauge pressure transducers using the principle of the Wheatstone bridge. Because of the need for pressure transmission to record a signal, a time delay is inherent and should be accounted for when assessing instantaneous pressure differences (e.g., gradients). Moreover, fluid-filled systems are influenced by various factors, including transducer height, patient position, catheter length, air bubbles, fluid frequency response/damping (e.g., catheter whip artifacts), and open connections.
These issues can be avoided by using micromanometer catheters that use high fidelity pressure sensors at the point of measurement (Millar Instruments, Houston, TX). Such systems can also be combined with special conductance catheters to provide simultaneous volume measurements, but their use is confined to research laboratories. These systems provide more sophisticated measures of ventricular performance such as contractility, diastolic function, and ventricular pressure-volume (PV) relationships.
The impact of respiration on intracardiac pressures should be considered. The dynamic changes in intrathoracic pressures associated with respiration are usually modest (e.g., −1 to 5 mm Hg) but can be quite dramatic (e.g., severe obesity, obstructive pulmonary disease) and will have a significant influence on intracardiac pressures. However, it is common in both clinical practice and investigation that mean pressure measurements rather than respirophasic ranges are reported, which can lead to important misinterpretations. For example, the pulmonary capillary wedge pressure (PCWP) averaged over the duration of the respiratory cycle may underestimate the true end-expiratory left ventricular end-diastolic pressure (LVEDP) and lead to an erroneous diagnosis of World Health Organization (WHO) group 1 rather than group 2 or mixed PH. It is optimal to manually measure end-expiratory pressures since the effects of negative intrathoracic pressures required for ventilation on intracardiac pressures are minimal at end expiration. A breath hold can be useful if done at end expiration as long as a Valsalva maneuver does not ensue.
RHC is generally safe, but its routine use in heart failure management should be tempered by complications associated with any invasive procedure. Serious complications, such as pneumothorax, pulmonary embolism, and pulmonary arterial rupture are rare. In the ESCAPE (Evaluation Study of Congestive Heart Failure and Pulmonary Artery Catheterization Effectiveness) trial, in-hospital adverse events were more common among patients in the RHC group compared with the clinical assessment group (22% vs. 11.5%; P = .04). Adverse events due to the pulmonary artery catheter (PAC) occurred in 4% (n = 9) of the RHC group. The most common complication was access site bleeding. There were no hospital deaths attributed to the PAC. Adverse events included PAC-related infection, catheter knotting, pulmonary infarction and hemorrhage, and ICD shocks. Pulmonary arterial rupture and RBBB (with subsequent complete heart block in the setting of a baseline LBBB) did not occur. Pulmonary artery (PA) rupture is rare in clinical practice, but risk factors include severe PH, anticoagulation, mitral valve disease, and advanced age.
Hemodynamic Waveforms
In clinical practice, it is difficult to measure LV volume throughout the cardiac cycle rendering the use of PV loops for clinical care impractical. The difficulty in obtaining PV loops has resulted in a dependence on pressure versus time rather than pressure versus volume measurements to assess hemodynamic status and ventricular performance.
Each chamber has a distinct waveform and reflects cardiac filling as well as ventricular ejection. Personal inspection of specific cardiac chamber waveforms can often convey important hemodynamic information that is not appreciated by a single measured value.
Right Atrium and Ventricle
The right atrial pressure (RAP) and waveform can provide simple yet important insight into a patient’s overall hemodynamic status. During normal respiration, RAP decreases with inspiration to the same degree as the drop in intrapleural pressure (usually <5 mm Hg) and generally reflects intrapericardial pressure in the absence of pericardial constraint. Lack of an inspiratory fall, or even rise in RAP with inspiration (e.g., Kussmaul sign), is indicative of right ventricle (RV) diastolic dysfunction and often RV overload ( Fig. 34.2 ). Steep y descents are also indicative of RV diastolic dysfunction ( Fig. 34.3 ). The steep y descent results from the rapid inflow required to fill a stiff RV in early diastole, particularly in the presence of an elevated RAP. Similarly, the “dip and plateau” appearance in the RV tracing indicates rapid, early diastolic filling and abrupt cessation of diastolic inflow as the LV and RV reach the point of pericardial restraint. Such findings are commonly found in conditions associated with RV dysfunction, including advanced heart failure with reduced ejection fraction (HFrEF), restrictive cardiomyopathies, heart transplantation, and RV infarction. A prominent v wave is noted in severe tricuspid regurgitation, regardless of chronicity ( Fig. 34.4 ). The central venous pressure may also be approximated by the venous pressure in a peripheral vein with appropriate establishment of the phlebostatic zero reference.



The RV is a low-pressure chamber in normal individuals. A small systolic gradient between the RV systolic pressure (RVSP) and PA systolic pressure (PASP) facilitates forward flow in this low-pressure system. The RV waveform can be distinguished from the PA waveform by the rise in pressure during diastole in contrast to the fall in pressure in the PA tracing. The rise in the RVSP over time, dP/dt, can be used to estimate the PA diastolic pressure (ePAD) since pulmonic valve opening will occur at the end of isovolumic contraction or maximum dP/dt ( Fig. 34.5 ). The ePAD will approximate the PCWP (e.g., within 2–3 mm Hg) as long as the pulmonary vascular resistance is normal. This strategy was used for the CHRONICLE device to provide a continuous estimate of the PCWP.

Pulmonary Capillary Wedge
A common concern in RHC is whether a true PCWP has been obtained ( Fig. 34.6 ). Fluoroscopy and a visible change in the waveform is typically used to confirm the PCWP position. Loss of the pulmonary arterial waveform and a change to a “flattened” appearance with attenuated deflections usually signifies the PCWP. A prominent v wave can also be seen with a true PCWP but can be easily confused with the PASP. The v wave of PCWP occurs well after the T wave of the ECG, whereas the peak systolic wave of PASP occurs before or within the T wave. Difficulty in distinguishing between PCWP and PASP is particularly problematic in patients with prominent v waves (e.g., acute severe mitral regurgitation, ventricular septal defects, and severe diastolic dysfunction).

Hybrid tracings between PCWP and actual pulmonary artery pressure (PAP) result in an overestimation of PCWP and should be suspected when the PCWP exceeds the PA diastolic pressure ( Fig. 34.7 ). Damping of the hemodynamic waveform should also be considered. Underdamping, which is often referred to as ringing or “whip” artifact, can lead to inaccurate pressure recordings and can be rectified by drawing a small amount of blood or contrast into the catheter to increase the viscosity of the fluid. In patients with severe right ventricular enlargement and dysfunction, caution must be used when it comes to interpreting an elevated PCWP or LVEDP. Right ventricular pressure overload from pulmonary artery hypertension (PAH) can result in elevated LVEDP due to impaired relaxation of the LV secondary to RV enlargement as well as pericardial restraint (see also later discussion). In this setting, LV diastolic dysfunction is a consequence and not independent of the primary abnormality (PAH).

If there is still uncertainty about the accuracy of the measured PCWP, it is best confirmed by other means. A common strategy is to use the O 2 saturation from a blood sample obtained from the PCWP position. In the true PCWP position, the O 2 saturation from the PCWP blood sample should have an O 2 saturation comparable to systemic arterial saturation (in the absence of intracardiac shunts). Wedge position can also be confirmed by cautious injection of contrast to document true wedging of the balloon. LVEDP should be directly measured if uncertainty exists as to the accuracy of the PCWP as a surrogate for left ventricular filling. In circumstances where accurate assessment of left atrial pressure (LAP) is critical, consideration should be given to directly measuring LAP across the atrial septum.
The PCWP waveform is similar to that seen in the left atrium with well characterized a and v waves, in addition to x and y descent ( c waves are less apparent in PCWP tracings). PCWP waveforms are also slightly damped and delayed compared to that seen in the LA because of transmission of the pressures through the lung parenchyma. As noted earlier, in the absence of increased pulmonary vascular resistance, PA diastolic pressure is similar to mean PCWP (e.g., within 2–3 mm Hg). If there is PH, the PA end-diastolic pressure will be markedly higher than PCWP. Furthermore, when pulmonary vascular resistance is increased by pulmonary venous hypertension or mitral stenosis, PCWP may overestimate LVEDP. PCWP may also underestimate LVEDP since PCWP is an intrathoracic pressure that is an indirect assessment of LVEDP and is not a direct measurement of LV filling pressure. In one study, PCWP was more than 5 mm Hg lower than LVEDP in about a third of cardiac patients. Therefore when it is critical to have a definitive measure of LV filling pressure, direct measurement of LVEDP is necessary.
The height of the v wave should be reported separately from the mean PCWP, particularly when it exceeds the height of the a wave by greater than 50%. The height of the v wave is determined by left atrial chamber compliance and distending blood volume. Although most commonly associated with acute severe mitral regurgitation (due to the sudden retrograde volume overwhelming the compliance of the left atrium), it is also characteristic of a large volume ventricular septal defect and can be seen in severe left ventricular diastolic dysfunction.
Large v waves augment the mean PCWP and can lead to overestimation in LAPs , as in Fig. 34.8 . In this setting, the mean a wave pressure should be reported, in addition to the mean PCWP and height of the v wave, to reflect the end-diastolic PCWP. Another approach is to use the onset of the QRS, complex to define the end-diastolic PCWP.

Measuring Cardiac Output
The two most commonly used methods for measuring cardiac output (CO) are the thermodilution and Fick methods. Both methods are limited by technical issues and physiologic assumptions. The indication-dilution technique is rarely used in contemporary clinical practice but forms the basis for the thermodilution method.
In the thermodilution method, a known volume of cold saline or dextrose is injected at a known temperature into the proximal port of the PAC. The subsequent change in temperature of this solution is measured by a thermistor at the distal end of the catheter. The change in temperature of the injectate allows measurement of the CO. If the temperature does not change markedly and remains close to the temperature at which it was injected, this reflects a high CO because there is insufficient time for the surrounding structures to transfer heat energy to the fluid. In contrast, if the CO is low, there will be ample time to transfer heat energy from the blood to the injectate before it passes the thermistor at the distal end of the catheter. In this setting, the temperature of the injectate will change markedly and will be reflective of a decreased CO. The CO is calculated using an equation that incorporates a calibration factor plus the volume, the specific gravity, and the temperature of the injectate along with the temperature and specific gravity of the blood. The CO is inversely related to the area under the thermodilution curve (e.g., change in temperature over time) with a larger area reflective of a lower CO. Accuracy of the CO measurement is improved by a greater difference between the temperature of the injectate and the temperature of the blood. The thermodilution technique overestimates flow in patients with low output states. Concern is frequently raised regarding the accuracy of thermodilution in patients with severe tricuspid regurgitation, but it remains remarkably useful even in this setting. A large retrospective analysis of more than 1200 patients who underwent RHC demonstrated modest agreement between thermodilution and Fick CO (using an assumed oxygen consumption) (r = 0.65), with 38% of patients having a difference of more than 20%. However, the thermodilution CO was associated with a greater mortality risk (thermodilution hazard ratio [HR] 1.71 [95% confidence interval (CI) 1.47–1.99], Fick HR 1.42 [95% CI, 1.22–1.64]) ( Fig. 34.9 ).

The Fick method is based upon the principle that oxygen (O 2 ) consumption equals the product of O 2 delivery rate (e.g., CO) and O 2 extraction (e.g., the difference in O 2 content between the arterial and venous circulation). Thus CO equals the ratio of O 2 consumption to O 2 extraction and is therefore directly proportional to O 2 consumption. Measurement of O 2 consumption requires specialized equipment (e.g., a metabolic cart) to measure the content of O 2 in expired air. Since most clinical laboratories lack the capacity to make this measurement, most clinical laboratories assume a uniform normalized O 2 consumption (e.g., 125 cc/min/m 2 ) for all patients. Corrections for metabolic state, age, and gender are generally not made, and errors in O 2 consumption can be as high as 50% since O 2 consumption varies widely between patients and clinical status. Such issues are compounded during exercise hemodynamics. Errors in the Fick method can also occur if the mixed venous O 2 content is inaccurately measured due to contamination of the pulmonary arterial sample with blood from the PCWP. Blood from the PA is recommended for accurate assessment of the mixed venous oximetry as it mixes the O 2 content from the superior vena cava, inferior vena cava, and cardiac veins. Blood samples from the superior vena cava and right atrium are often used as surrogates for the mixed venous O 2 , but may not be adequate estimations in patients with sepsis, shunts, fistulas, and normal cardiac index. Peripheral venous oximetry can also be used to follow trends in CO, assuming oxygen consumption is relatively constant over time. Inaccurate assessment of hemoglobin content will also lead to measurement errors, and simultaneous measurement of hemoglobin concentration should be obtained if possible. If O 2 consumption is accurately measured, the Fick method is more accurate than the thermodilution method in patients with low CO.
Hemodynamics of Heart Failure With Reduced Ejection Fraction
The hemodynamic interaction between the left ventricle and the vascular system (e.g., ventricular-vascular coupling) for any degree of intrinsic myocardial contractility is best described with PV loops. In a PV loop, load-independent contractility is characterized by the slope of the end-systolic pressure-volume relationship (ESPVR) curve, or end-systolic elastance (Ees), which is also influenced by chamber size. Vascular afterload is characterized by arterial stiffness or elastance (Ea) and determined from the negative slope of the line through the end-systolic and end-diastolic volumes ( Fig. 34.10 ); this measurement integrates the resistive and pulsatile components of afterload and is heart-rate dependent. Ejection fraction, systolic blood pressure, stroke volume, and stroke work can all be derived from end diastolic volume (EDV) and these elastances.

The ratio of these two measurements represents ventricular-arterial coupling (Ea/Ees) and describes the “matching” of vascular load to myocardial function. Experimental studies suggest an optimal Ea/Ees ratio is 0.6 to 1.2, which maximizes the efficient transfer of blood from the heart into the systemic circulation. In patients with HFrEF, this ratio is increased since ventricular elastance (Ees) is depressed from the underlying cardiomyopathy and arterial elastance (Ea) is increased from neurohormonal activation and vasoconstriction. This afterload “mismatch” has prognostic implications independent of EF and can be a target for therapy (see later discussion). In a recent noninvasive multicenter study of HFrEF patients, an Ea/Ees ratio greater than 2.34 was found an independent risk factor of all-cause mortality, cardiac transplantation, or VAD implantation (HR 2.1, 95% CI 1.3–3.3).
It is important to note that systemic blood pressure and systemic vascular resistance (SVR) do not adequately describe afterload in a pulsatile system. In clinical practice, the resistive load is described by the SVR ([MAP—CVP]/CO); the pulsatile load is usually not described. The pulsatile load can be quantified by the total systemic arterial compliance (C a ) (Stroke Volume Index/aortic pulse pressure). Because the aorta provides the majority of this pulsatile load, central pulsatile load (or characteristic impedance, Zc = change in pressure/change in flow in early systole) better characterizes the work that must be overcome by the LV during ejection and is elevated in HFrEF. Although not practical for routine clinical use, the effective arterial elastance (Ea = ESP/SV, where ESP, end systolic pressure; SV = stroke volume) combines the resistive and pulsatile components of LV afterload and best describes the impact of ventricular-vascular coupling to LV performance.
In HFrEF, vasodilation can dramatically improve stroke volume since the reduced contractile state is particularly afterload sensitive and characterized by an increased Ea/Ees ratio (e.g., afterload “mismatch”). Vasodilator therapy takes advantage of the inverse relationship between CO and impedance. In HFrEF, the afterload sensitive nature of the failing LV is reflected by the relatively flat ESPVR, for example, shallow Ees. This flat ESPVR accounts for the marked improvement in stroke volume that occurs with vasodilator therapy without a significant drop in blood pressure ( Fig. 34.11 ).

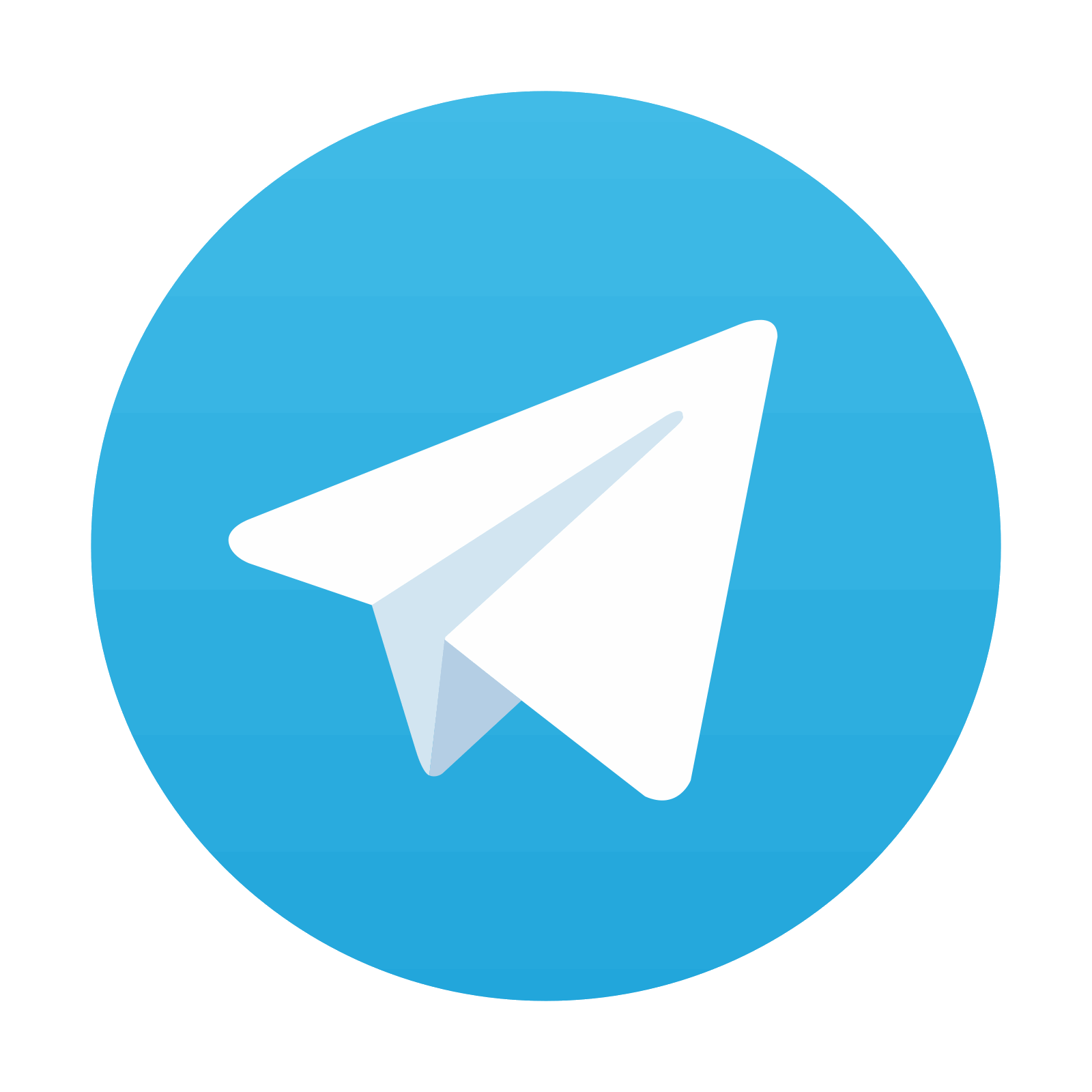
Stay updated, free articles. Join our Telegram channel
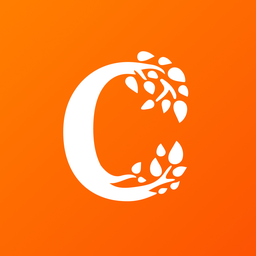
Full access? Get Clinical Tree
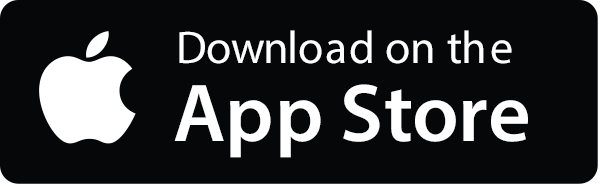
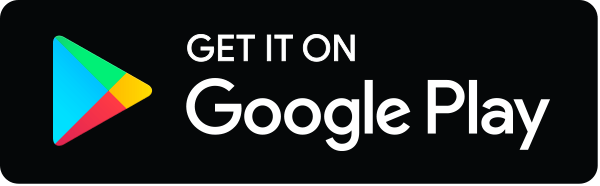
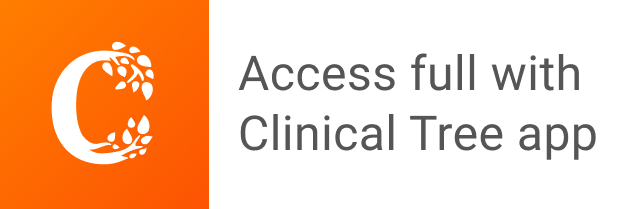