Renal artery stenosis (RAS) is the main cause of “secondary” arterial hypertension. RAS is found in 0.5% to 5% of hypertensive patients. RAS is most often atherosclerotic in nature and less frequent due to fibromuscular hyperplasia.1 The prevalence of RAS is approximately 2% in unselected patients but reaches 40% in older patients or in patients with multiple risk factors for atherosclerosis or with documented atherosclerosis in other vascular territories.2,3 Due to improvements in vascular imaging by ultrasound, magnetic resonance imaging, computed tomography, and angiography and due to the larger proportion of elderly patients undergoing coronary angiography, the finding of a RAS on angiography is a frequent occurrence. “Drive-by” renal angiograms are frequently performed during coronary angiography procedures. However, as is the case in the coronary arteries, the relationship between anatomic findings and the functional repercussion of a given stenosis is poor.4
Thus, stenoses of the main trunk of the renal arteries are frequent and easy to identify, but their causative role in clinical manifestations is difficult to evaluate, and it is difficult to predict the effect of renal stenting
Renal artery stenoses contribute to arterial hypertension and/or to ischemic nephropathy.
Much modern thinking about the physiopathology of renovascular hypertension is influenced by the classical 1-kidney-1-clip animal model (analogous to a bilateral RAS)5 and the 2-kidneys-1-clip model6 (analogous to the unilateral RAS). A simplified scheme illustrating the pathophysiology of renovascular hypertension is shown in Figure 7-1. When the perfusion pressure decreases in the renal artery, especially in the afferent renal artery, an upregulation of renin production takes place in the juxtaglomerular apparatus. The decrease in glomerular filtration pressure will be partially counteracted by a constriction of the efferent artery. The activation of the renin-angiotensin system resulting in angiotensin II–mediated vasoconstriction is a central component to this process. In contrast, the nonstenotic kidney, subjected to higher perfusion pressure, responds by an increase in the excretion of sodium (“pressure natriuresis”) that will tend to lower the pressure. This decrease in systemic pressure will in turn decrease the perfusion pressure in the stenotic kidney and further stimulate the release of renin. Elevated levels of angiotensin II induce vasoconstriction and trigger the secretion of aldosterone. The latter enhances sodium reabsorption in proximal and distal tubules. Thus, renovascular hypertension is mediated initially by elevated plasma renin, although in case of sustained hypertension, plasma renin activity will tend to decrease. Renovascular hypertension fundamentally depends on the presence and magnitude of a pressure drop due to the stenosis.
The most common cause of renal insufficiency in patients with RAS is the association of the RAS to parenchymal disease. In contrast, the occurrence of renal failure solely due to a stenosis in the trunk of the renal artery is exceptional in unilateral RAS and rare in bilateral RAS in the absence of parenchymal disease or nephrotoxic medications. In rare cases, when bilateral stenoses are critical, the perfusion pressure may become so low that the renal tissue becomes ischemic, which in turn might lead to necrosis and fibrosis. In addition, in patients with bilateral RAS or RAS in a solitary kidney, the maintenance of a sufficient glomerular filtration pressure by vasoconstriction of the efferent artery is highly dependent on angiotensin II. When angiotensin-converting enzyme inhibitors are given, the efferent arteriolar tone is no longer maintained, and glomerular filtration rate decreases, as in patients with cardiac failure who are sodium depleted.
Like most organs, the myocardium and the renal parenchyma are perfused by large arteries opposing no or very little resistance to flow. These arteries are often the seat of atherosclerotic narrowing. Smaller arteries and arterioles penetrate the tissue, are the site of the largest resistance, and give rise to feeding vessels, the capillaries. Due to their fundamentally different functions (pump function for the myocardium and filter function for the kidney), the consequences of a narrowing on the main arteries are almost opposite of the consequences on pressure and blood flow.
The main “goal” of the myocardium is to maintain constant blood flow to allow enough oxygen and nutrients to be supplied to the contracting myofilaments. Cardiac oxygen requirements are met thanks to a high level of oxygen extraction by the myocardium. This explains the low oxygen saturation in the coronary sinus (20%). The main mechanism to increase oxygen supply is to increase absolute myocardial flow. Therefore, a gradually increasing stenosis in the epicardial arteries induces a decrease in coronary driving pressure and will be compensated by a vasodilation of the microvasculature. This mechanism, often referred to as autoregulation, maintains a constant total resistance to flow, thus allowing a normal myocardial flow (“perfusion”). This vasodilation is mainly mediated by adenosine A21 receptors. The regulation is mainly based on a local mechanism.
In contrast, renal arteries deliver more oxygenated blood to the kidney than needed for metabolic demands. Renal metabolic requirements are met with 10% of normal blood flow. This translates into a high renal venous oxygen saturation (80%). The main “goal” of the kidney is to maintain glomerular filtration constant. A decrease in the pressure in the main trunk of the renal artery, and thus in the afferent renal artery, will be compensated by an increase in the tone of the efferent renal artery, thus maintaining glomerular filtration pressure constant. This vasoconstriction is the result of an immediate local myogenic reflex and a delayed systemic activation of the renin-angiotensin system. The vasoconstriction is largely based on the activation of adenosine A1 receptors. The administration of adenosine in the coronary artery leads to vasodilation, whereas adenosine induces a potent vasoconstriction in the renal circulation (Fig. 7-2).
FIGURE 7-2
Difference between renal artery and coronary flow response to adenosine (ADO) administration expressed in centimeters per second. A. Renal artery vasoconstriction induced by intrarenal artery adenosine bolus administration (arrow). B. Coronary vasodilatation induced by intracoronary adenosine bolus administration (arrow). LAD, left anterior descending artery.

In this teleologic view of physiology, one might consider that the myocardium tends to preserve flow at the cost of pressure, whereas the kidney tends to maintain pressure at the cost of flow.
Invasive assessment of RAS mainly encompasses angiography, Doppler flow velocity, and pressure measurements.
Recognition of the frequent association of atherosclerosis in the coronary and renal arteries has led to an increase in “drive-by” renal angiograms performed by cardiologists “on their way to work.” The additional contrast burden can be limited to 10 to 20 mL, the image quality is pristine, and the specificity is higher than that with duplex echocardiography or magnetic resonance angiography.7 As a result, the number of RASs detected in patients with arterial hypertension has increased, leaving unanswered the conundrum of the causality of these findings. Drive-by angiograms have brought more questions than answers. It is now well recognized that the relation between percent diameter stenosis and functional assessment of these lesions is poor, even for stenoses of more than 50% of diameter reduction (Fig. 7-3).4 Thus, invasive angiography is the most precise tool for the identification of RAS but does not give any clue about the potential renal angioplasty to reverse the hypertension or the loss of kidney function. Intravascular ultrasound–derived assessment of the luminal dimension does not seem to add to the utility of a morphologic approach8 (Fig. 7-4).
FIGURE 7-3
Plot of individual values of distal coronary pressure to aortic pressure (Pd/Pa) ratio versus percent diameter stenosis. The vertical line indicates the cut-off value for hemodynamic significance based on invasive pressure gradient measurement. The horizontal line corresponds with the cut-off value based on renal angiography (50% diameter stenosis).

By noninvasive Doppler ultrasonography, it is possible to derive the renal resistance index, which is defined as follows: (1 – [end-diastolic velocity/peak systolic velocity]) × 100. Structural alterations in smaller renal arteries or arterioles distal to the stenosis induced by longstanding hypertension may be one possible reason for the poor response to renal angioplasty. Such hypertension may cause nephrosclerosis9,10,11,12 and glomerulosclerosis13 and lead to increased vascular resistance in both the stenotic and nonstenotic kidney. It was shown that when the value of the renal resistance index exceeds 80, renal angioplasty is unlikely to improve renal function or arterial hypertension.14 Similarly, the reactivity of the distal renal arterial bed was tested with invasive intra-arterial Doppler wires in patients with essential hypertension but no RAS.15 These authors showed that nitrates induced a significant dilatation of the main renal artery and an increase in blood flow velocities, resulting in a 44% increase in absolute renal blood flow. Papaverine was shown to have no effects on the main renal artery but increased renal blood flow by 60% by vasodilation of the parenchymal arteries. More importantly, there were marked interpatient differences in the response to papaverine, suggesting that in some patients with essential hypertension a functional abnormality exists in the renal microcirculation. To the best of our knowledge, the usefulness of invasive renal flow velocity measurements has not been studied in the setting of RAS.
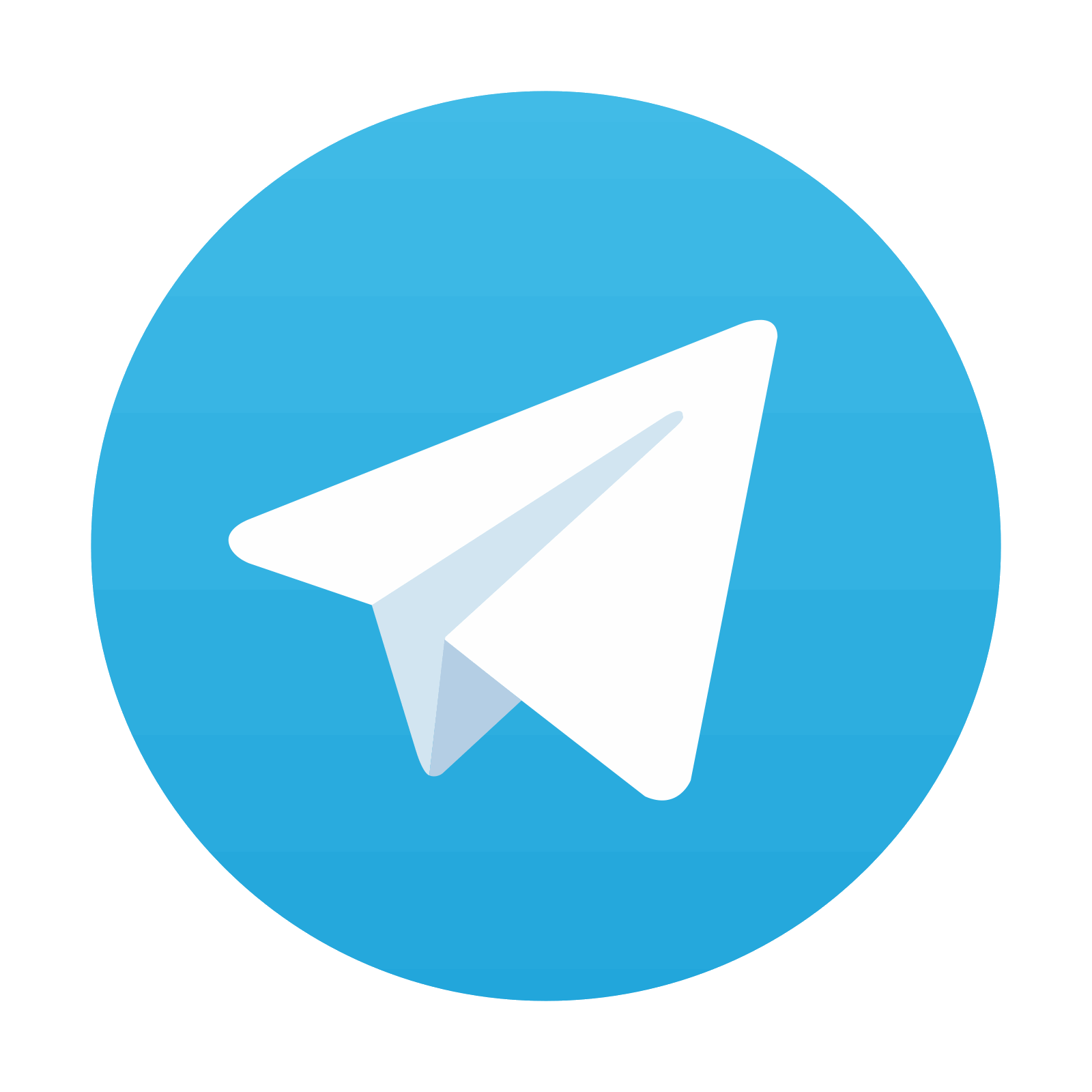
Stay updated, free articles. Join our Telegram channel
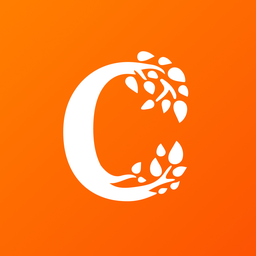
Full access? Get Clinical Tree
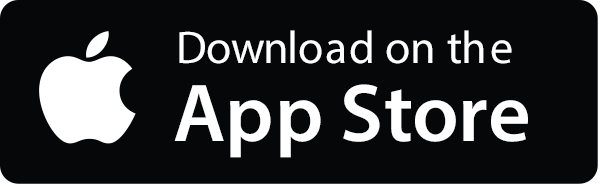
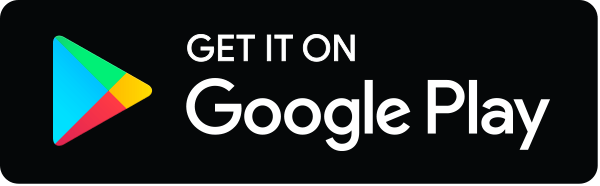