Hemodilution and Priming Solutions
John R. Cooper Jr.
N. Martin Giesecke
HISTORICAL PERSPECTIVE
Historical perspective is particularly important in assessing the progression of techniques for hemodilution and how they affect the physiology of extracorporeal circulation and the development of related complications. John Gibbon (1), who developed the first heart-lung machine and performed the first successful surgery using cardiopulmonary bypass (CPB), envisioned a perfusion technique employing a prime of normal composition (i.e., blood) with “normal” flow rates (70-80 mL/kg/min) and normal blood pressure. When Gibbon withdrew from this area of research, Kirklin et al. (2) continued Gibbon’s original methods.
Lillehei et al., first in procedures with controlled cross-circulation (3) and then with CPB (4), used significantly lower flow rates (30-35 mL/kg/min) than did Gibbon. This modification (based on the “azygous flow principle”) (5) was derived from the observation that dogs could survive when both the superior and inferior vena cavae were occluded, because the azygous venous drainage of dogs, unlike that of humans, still provided venous return to the heart on account of its more central confluence with the superior vena cava. In humans, this amount of flow (10% of normal cardiac output) proved adequate for survival for up to 1 hour. This observation was rapidly accepted and applied to the practice of CPB by many of those who perceived the harm to blood elements, as well as the excessive suctioning that results from the use of high flows. Hypothermia was added to many high-flow-rate and most low-flow-rate techniques to “protect” various organs, although Lillehei’s (3) original cross-circulation procedures were carried out at normothermia. Despite lower flow rates, the priming solution for the extracorporeal circuit with oxygenator remained the same—whole blood, which was either freshly drawn and heparinized or collected into citrated bags, but as fresh as possible.
Around 1960, spurred by animal research and the occasional emergent clinical experience with asanguinous prime, surgeons began electively using crystalloid solution or plasma-expanding colloids to reduce or eliminate blood from the prime (Table 16.1) (1,2,3,4,6,7,8,9). Hypothermia was now increasingly applied to protect organs from the “adverse effects” of not only low flow but also hemodilution. Nevertheless, the technique of hemodilution gained increasing popularity, and currently it is used almost universally.
TABLE 16.1. Historical perspective of priming solutions and use of hemodilution during cardiopulmonary bypass | |||||||||||||||||||||||||||
---|---|---|---|---|---|---|---|---|---|---|---|---|---|---|---|---|---|---|---|---|---|---|---|---|---|---|---|
|
The following discussion pertains to short-duration, clinical CPB used to aid cardiac surgery. Applicability of hemodilution to longer CPB support times is discussed elsewhere.
IMMEDIATE BENEFITS OF HEMODILUTION
A main reason for using asanguinous primes was to reduce the severe strain placed on hospital blood banks by the use of whole blood for priming CPB circuits. The need for at least two units of whole blood—and possibly as many as five or six, just for use as prime in each patient—often caused severe logistic problems for physicians trying to run an active cardiac surgical service. Many cases were postponed for lack of the correct type or amount of blood. Crystalloid primes also
improved access to CPB as an emergency procedure by reducing the time and resources required for having a usable circuit available (Table 16.1) (1,2,3,4,6,7,8,9).
improved access to CPB as an emergency procedure by reducing the time and resources required for having a usable circuit available (Table 16.1) (1,2,3,4,6,7,8,9).
Another immediate but unforeseen clinical benefit of crystalloid primes was improved oxygenation during CPB. The types of bubble oxygenators widely used in the 1960s and early 1970s produced a high proportion of relatively large bubbles. Compared with the smaller, more numerous bubbles produced by later oxygenators, these larger bubbles were relatively inefficient for the transfer of oxygen, in part because of their lower ratio of surface area (where the gas transfer occurs) to volume, and in part because of the relatively smaller number of bubbles in a given volume of blood relative to the number of red blood cells. Hemodilution, especially in patients with polycythemia, which commonly accompanies congenital heart disease, produced an absolute reduction in the number of red blood cells and increased each cell’s likelihood of exposure to an oxygen transfer surface (bubble). Arterial oxygen tension during CPB was generally elevated, and additional crystalloid was given to maintain hemodilution if oxygen tension started to fall with diuresis.
A special impetus to the development of asanguinous prime techniques was provided by members of the Jehovah’s Witness faith, who totally refuse to accept transfusions of blood or blood products, although they will allow their own blood to circulate outside their bodies, as long as fluid continuity is constantly maintained in the CPB circuit. The use of asanguinous priming techniques has permitted cardiac operations, including transplantation, to be performed in more than 1,000 Jehovah’s Witnesses at the Texas Heart Institute alone (10,11). These patients constitute a unique “control” group for comparison with patients who undergo other techniques of blood conservation.
PHYSIOLOGIC CONSEQUENCES OF HEMODILUTION
Rheology of Blood
A detailed review of the rheologic properties of blood is beyond the scope of this chapter. However, some basic definitions and concepts are required, because the changes in blood rheology induced by hemodilution are central to any discussion of hemodilution physiology. Exhaustive reviews may be found elsewhere (12,13).
A force that is applied to an area of a liquid confined between two plates and that is sufficient to set the liquid in motion is known as shear stress. The velocity at which the liquid moves in proportion to the separation of the plates is known as the velocity gradient, or shear rate. The shear stress is proportional to the shear rate, and the coefficient of proportionality is the fluid viscosity (Eq. 1).

For most uniform fluids, such as water, viscosity is constant; these are known as Newtonian fluids. The viscosity of blood is not constant; rather, it depends on the shear rate when blood is flowing (Fig. 16.1). Therefore, blood is a non-Newtonian fluid. Lower shear rates are associated with higher viscosity, because the cellular elements and plasma proteins tend to aggregate, form rouleaux, and resist flow. This aggregation at low shear rates primarily results from intracellular bridging by fibrinogen; as flow rates increase, these bridges disintegrate. Non-Newtonian behavior of blood is also influenced by the effective cell volume (14). When solid particles (cells) are added to a fluid, they influence viscosity not only by their absolute presence but also by their effect on the surrounding fluid. The effective cell volume is the cell volume plus the surrounding fluid that behaves as though it were a part of the cell—much like the earth and its atmosphere. Red blood cells are deformable, and as shear rates increase, the cells tend to form ellipsoid shapes, with their major axes aligned with the direction of flow. This latter effect, combined with the disaggregation of red blood cells, causes blood to behave in a more Newtonian manner at higher velocity gradients.
One further concept about the behavior of fluids is important. The amount of shear stress that is necessary to cause a stationary Newtonian liquid to begin moving is zero or nearly zero. As a non-Newtonian substance, blood, because of its cellular geometry and aggregation, must be induced to flow by a force known as the yield stress. At low shear rates, the yield stress represents part of the viscous resistance and depends on both fibrinogen and hematocrit (15).
Circulatory Effects
Both organ blood flow and total cardiac output are directly proportional to the perfusion pressure and inversely related to total peripheral resistance (Eq. 2A). This resistance to flow
is proportional to vascular resistance and the viscosity of the perfusate (Eq. 2B).
is proportional to vascular resistance and the viscosity of the perfusate (Eq. 2B).


These relations are often specifically expressed in the Hagen-Poiseuille equation (Eq. 3):

where Q equals flow, P1dP2 equals the pressure drop along a tube of radius R and length L, and µ equals viscosity. For measuring blood flow, this equation is limited, because it is accurate only for assessing the laminar flow of Newtonian liquids in long tubes with rigid walls. However, the equation does serve to further emphasize the influence of viscosity and vascular geometry on flow. In humans, the aorta and larger vessels provide little impedance to blood flow; most of the vascular resistance comes from the smaller vessels: the arterioles, capillaries, and venules. As the vessel diameter decreases, the shear rate also decreases, and because blood viscosity is inversely related to the shear rate, viscosity increases as flow decreases. As a result, peripheral resistance also increases. Flow is lowest and viscosity highest in the postcapillary venules (16).
These physiologic effects are potentially quite harmful if CPB is performed without considering viscosity. In most centers, modern CPB involves the use of flow rates that are somewhat lower than those of “normal” blood: 50 mL/kg/min or 2.0 L/m2/min. Frequently, hypothermia is also used if flow rates are further reduced to provide a bloodless operative field. In addition, hypothermia is commonly used to augment myocardial and cerebral protection. Reductions in both flow rate and perfusion pressure would tend to increase viscosity and, thereby, peripheral resistance. In turn, this would decrease tissue perfusion. Hemodilution works to limit the adverse effects of CPB by significantly reducing blood viscosity during bypass. Because there is a direct relationship between viscosity and hematocrit (12) (Fig. 16.1), a reduction in hematocrit produces a marked decrease in total resistance and an increase in tissue perfusion (Eq. 2B). For example, in canine experiments, a decrease in hematocrit from 42% to 25% produces a 50% increase in flow at the same pressure (16). Extremes of hemodilution (hematocrit <10%) permit blood to act as a Newtonian fluid (17).
Clinically, the most noticeable effect of hemodilution is a marked reduction in the perfusion pressure at initiation of CPB. Gordon et al. (12) showed that when hemodilution is used to decrease the hematocrit and when the flow rate on CPB is held constant, the perfusion pressure decreases in direct proportion to the change in viscosity (Fig. 16.2). Additionally, Guyton and Richardson (18) found that hemodilution passively increases venous return in dogs; this is probably attributable to the marked increase in flow in the small vessels, especially the postcapillary venules (Fig. 16.3). Cooley et al. (6) noted this same phenomenon during CPB in humans.
The addition of induced hypothermia to CPB further influences the rheologic behavior of blood. A temperature reduction decreases flow by inducing direct vasoconstriction and increasing viscosity (19) (Fig. 16.4). However, this relationship is not as direct as that of hematocrit and viscosity. For example, a temperature decrease of 10°C causes an approximately 20 to 25% increase in viscosity. Of course, this adverse effect of hypothermia is partially or completely offset by the planned reduction in oxygen consumption.
Organ blood flow during hemodilution reflects the interplay of all these concepts. In animals with progressive normovolemic hemodilution to a hematocrit of 19%, oxygen tension has been shown to increase in the skeletal muscle, liver, pancreas, small intestine, and kidneys (16). Cerebral blood flow during hemodilution also has been shown to increase by 50% to 300% when compared with prebypass levels (20,21). Decreases in the hematocrit correlate with increases in cerebral blood flow velocity, as measured by transcranial Doppler (22). However, because cerebral flow is also significantly influenced by local autoregulation, changes in carbon dioxide tension (PCO2), and extremes of perfusion pressure (23), absolute statements about the regional benefits of hemodilution are difficult to make. Nevertheless, hemodilution, in general, appears to have a salutary effect.
An exceedingly important consequence of hemodilution during CPB is an “uncoupling” of the normal relationship between perfusion pressure and blood flow. Therefore, perfusion pressure cannot serve as a marker of adequate flow, because pressure is a function of two variables: flow and viscosity. This pivotal concept bears directly on both the incidence and the causes of complications associated with the use of CPB (discussed later).
Hemodilution, Anesthetic Agents, and Adjuvants
Anesthetic agents and vasoactive adjuvant drugs can influence vascular geometry during CPB and thereby alter vascular resistance; these effects are independent of the effects related to hemodilution. Hemodilution can also alter the pharmacokinetics and pharmacodynamics of drugs, principally by dilution and decreased protein binding. Although the total drug concentration is usually decreased during CPB because of dilution, the amount of free active drug probably changes little because of decreased protein binding (24). During CPB, drug kinetics are also influenced by temperature, pH, oncotic pressure, and, sometimes, sequestration in the CPB circuit or the excluded vascular beds (i.e., the pulmonary vascular bed). These multiple factors make the study of drug activity during CPB interesting but exceedingly difficult (25).
Considerations in Oxygen Transport
Hemoglobin Physiology
Oxygen transport is the movement of molecular oxygen from the atmosphere to the cellular mitochondria. This movement depends on oxygen availability, cardiac output, hemoglobin, tissue perfusion, and the tissues’ ability to extract oxygen. Hemoglobin provides a tremendous physiologic advantage to the process of oxygen transport, in that relatively larger volumes of oxygen can be brought to the tissues by oxygen bound to the hemoglobin molecule than could be transported in a simple solution in the plasma.
Reduction of hemoglobin concentrations to below normal levels still permits adequate oxygen transfer. In fact, when oxygen transport is expressed as the product of cardiac output and oxygen-carrying capacity and is plotted against changes in the hematocrit (Fig. 16.5), maximal delivery occurs at a hematocrit of approximately 30%; more importantly, delivery decreases only approximately 10% below normal between hematocrits of 20% and 50% (26). This preservation of oxygen delivery obviously depends on a compensatory increase in the cardiac output at lower hematocrit ranges. Hemoglobin concentrations are reduced as a normal consequence of CPB with hemodilution. Without an increase in the cardiac output, such a reduction will decrease oxygen delivery, but this is usually well tolerated clinically. The lower limit to which hemoglobin can be reduced under any circumstance without a compromise in patient safety is not absolutely clear (as discussed later). On the basis of the previously described oxygen transport concepts, a hemoglobin concentration of 10 g/dL was traditionally (and institutionally) accepted as adequate for patients undergoing noncardiac surgery; however, it has
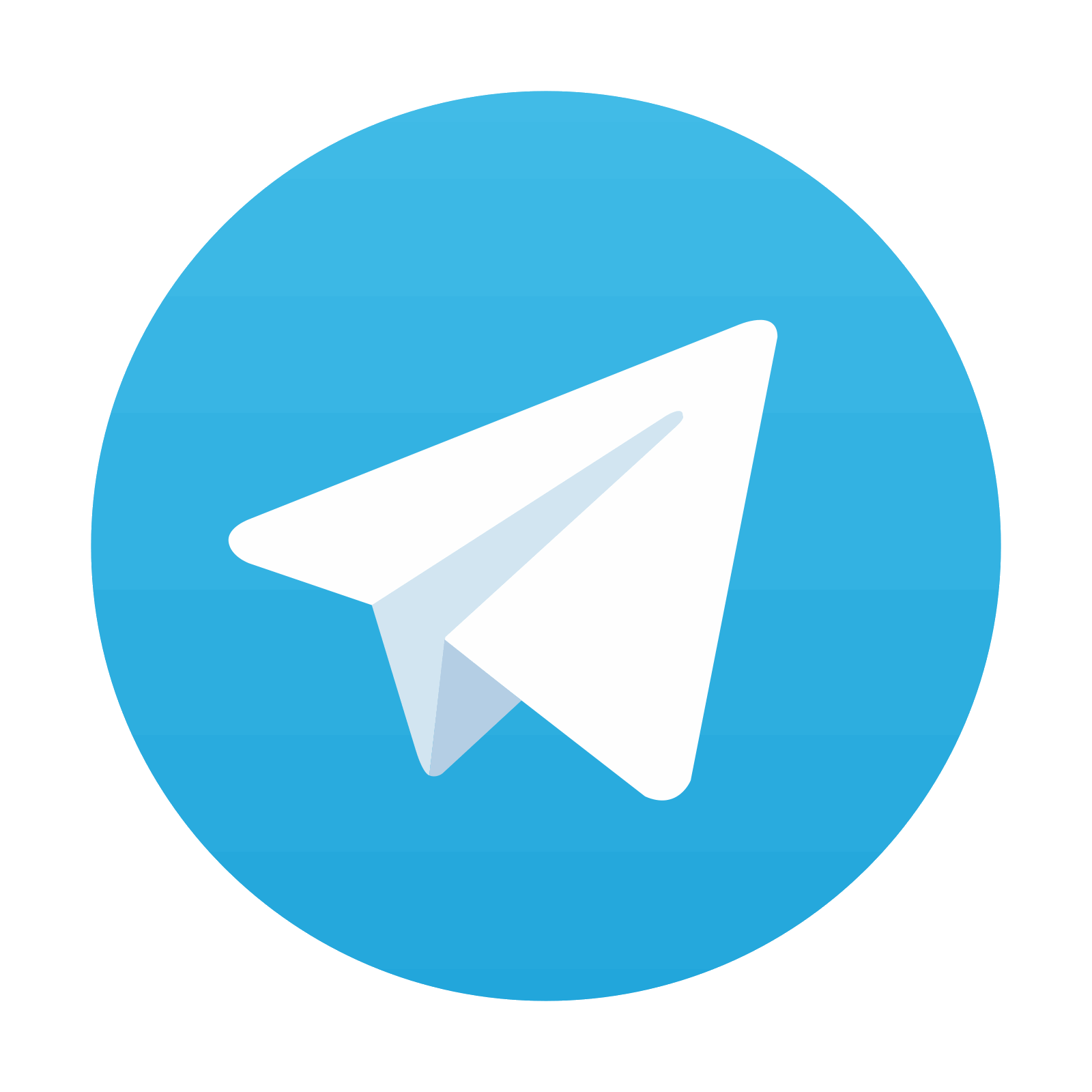
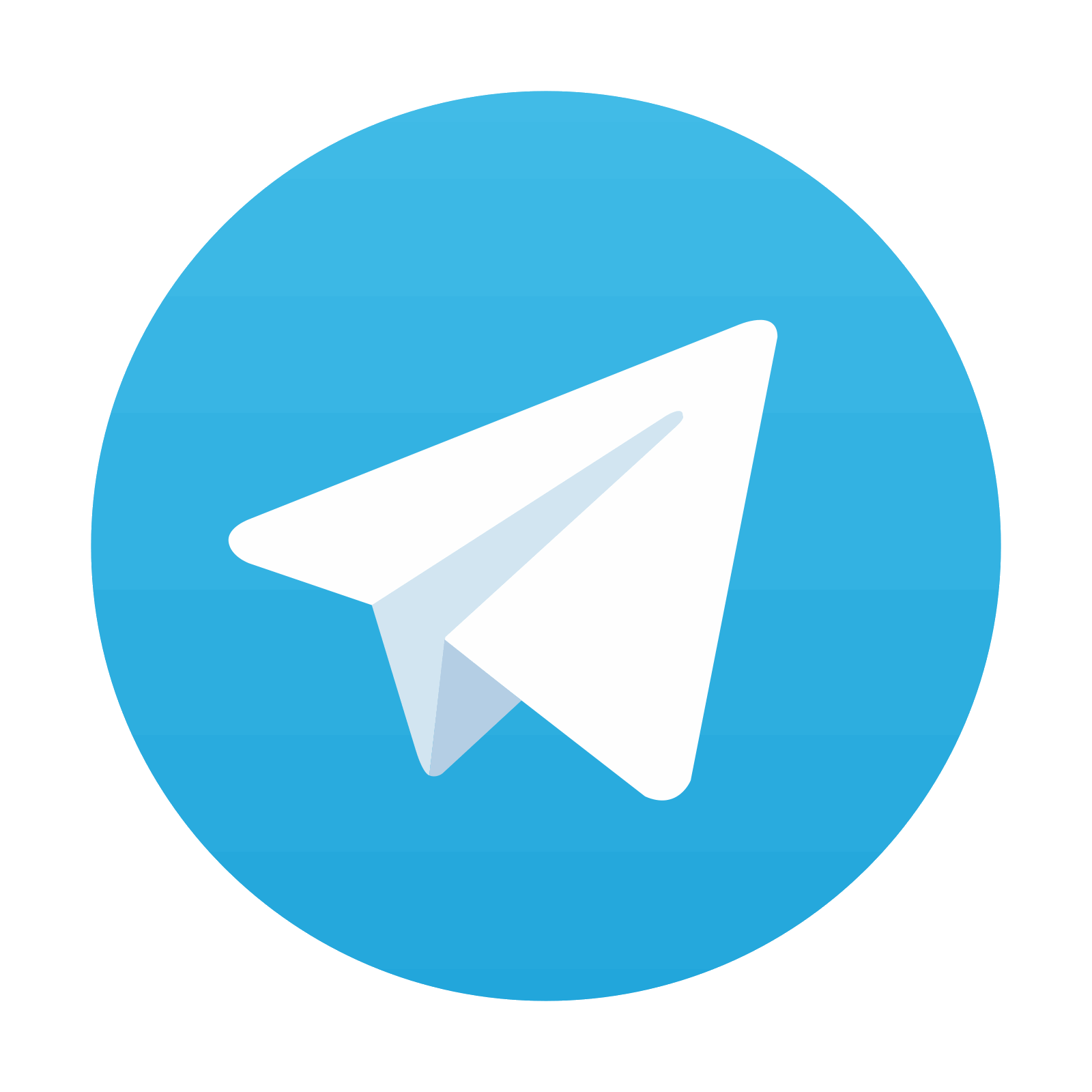
Stay updated, free articles. Join our Telegram channel
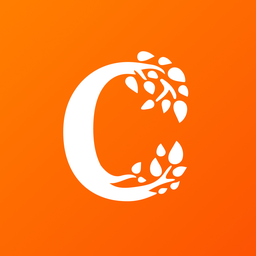
Full access? Get Clinical Tree
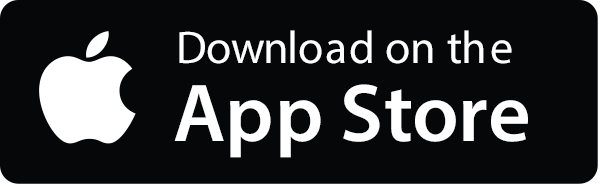
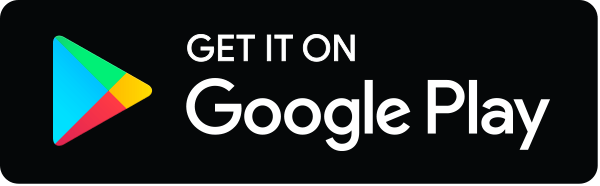
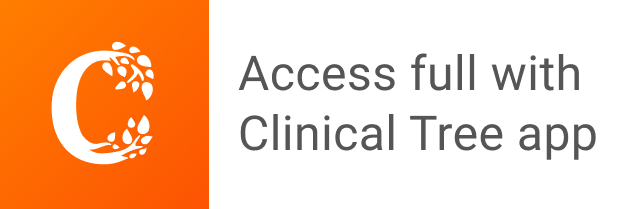