(1)
Project-team INRIA-UPMC-CNRS REO Laboratoire Jacques-Louis Lions, CNRS UMR 7598, Université Pierre et Marie Curie, Place Jussieu 4, 75252 Paris Cedex 05, France
Abstract
The heart lodges in the mediastinum. It is usually situated in the middle of the thorax with its largest part in the left side; its apex points toward in the inferior and left direction. However, sometimes it resides on the right thoracic region (dextrocardia situs inversus totalis).
The heart lodges in the mediastinum. It is usually situated in the middle of the thorax with its largest part in the left side; its apex points toward in the inferior and left direction. However, sometimes it resides on the right thoracic region (dextrocardia situs inversus totalis).
The heart is made of 2 synchronized left and right pumps with a common wall, the septum between the left and right atria at its upper part and left and right ventricles at its lower part. These twin pumps in series are composed of 2 chambers — upstream atrium and downstream ventricle — to adapt to the pressure difference between the low-pressure, upstream, venous compartment and high-pressure, downstream, arterial bed.
Under sedentary conditions, atria are minor contributors to blood pumping. During exercise, atrial contraction rises to increase the amount of blood that fills the ventricles (atrial kick). This blood-pumping reserve falls during aging as well as during atrial fibrillation.
Arteries leave the cardiac pump, without relation with the blood gas content; veins run toward atria. The left ventricle propels blood through the systemic circulation that carries arterial oxygenated blood under high arterial pressure; the aorta is the arterial trunk that receive blood from the left ventricle during the ejection phase of its contraction. The right ventricle expels blood through the pulmonary circulation that transport arterial deoxygenated blood with a lower arterial pressure; the pulmonary arterial trunk corresponds to the exit of the right ventricle.
Cardiac muscle cells, or cardiomyocytes (CMC; Chaps. 5), are joined both directly and indirectly via fibers of the extracellular matrix to form muscular bundles (myofibers) connected to neighboring coronary vessels and nerves (Vol. 6 –Chaps. 2. Anatomy of the Cardiovascular System and 4. Cardiovascular Physiology). These myofibers form muscular layers within the heart wall thickness that are characterized by their orientation (Sect. 5.9), which influences spreading of the electrochemical command (cardiomyocyte depolarization) wave and cardiac wall deformation associated with cardiomyocyte contraction.
Diverse cell populations are structural determinants of heart function. Cardiomyocytes represent the main cell population ( ∼ 75%) in the myocardium. Cardiac fibroblast is the main producer of the extracellular matrix with its collagen and elastin bundles and interconnected basement membranes.
Vascular cells are close to cardiomyocytes to efficiently supply nutrients. Parasympathetic and sympathetic nerve fibers extend within the myocardium. The right and left vagus nerves primarily innervate the sinoatrial and atrioventricular nodes responsible for the genesis and proper propagation of the electrochemical wave, respectively. Sympathetic nerves are located inside the atria and ventricles.
6.1 Cardiogenesis and Cardiac Remodeling
The heart is the first organ to form during embryogenesis. This first functional organ has a mesodermal origin. Growth and remodeling of the embryonic myocardium adapt dynamically to fit the changing needs of a developing body. Its contraction supports heart shaping via afeedback between the hemodynamic stress field and cardiac cells.
Soon after gastrulation, the heart starts to develop from cells of the mesoderm (Chaps. 11). The main stages of cardiogenesis are briefly described in Table 6.1. In vertebrates, the heart is initially built from the fusion of the 2 endocardial tubes that arise in the splanchnic mesoderm. The resulting primitive heart tube at the ventral midline undergoes a series of movements and remodelings to form the chambered organ. Disturbances of cardiogenesis cause cardiac malformations.
Table 6.1
Main stages of cardiac development. The precardiac mesoderm forms a primitive tubular heart that starts beating at about 3 weeks of gestation in humans. The heart maintains its pumping function despite its continuous remodeling into a 4-chamber organ. The heart has an asymmetric structure with anterior–posterior, cranial–caudal, and left–right polarity.
Primary heart fields | Two mesoderm lateral regions that appear shortly |
after gastrulation with precursors of cardiac | |
lineages (early myocardium) | |
Cardiac crescent | Merging of heart fields at the embryo midline |
Linear heart tube | Endothelial tube surrounded by a single layer |
of myocardial cells | |
Looped heart | Tube elongation and looping with gain of progenitors |
from the secondary heart field | |
Atrioventricular canal | Tube remodeling with a segment between atrial |
development | and ventricular regions |
Endocardial cushion formation | Outflow tract and atrioventricular cushions, i.e., |
precursors of heart valves; epithelial-to-mesenchymal | |
transition of endocardial cells | |
Heart wall trabeculation | Myocardium differentiation into 2 layers, an outer |
compact zone and an inner trabeculated zone; | |
coordinated proliferation and differentiation | |
of myocytes; crosstalk between trabecular myocytes | |
and endocardial cells | |
Chamber and outflow | Formation of 4 heart chambers and aorta |
tract septation | and pulmonary artery |
Markers of initial cardiac differentiation includeHeart and neural crest derivatives expressed HAND1 and HAND2 transcription factors, or class-A basic helix–loop–helix proteins bHLHa27 and bHLHa26, T-box transcription factorTBx5, as well as myosin regulatory light chain MLC2.1
Among the first features of anteroposterior patterning, atrial (aMHC1) and ventricular (vMHC1) myosin heavy chains are restricted to the anterior and posterior poles of the heart tube, respectively.
Two myosin heavy chain genes are expressed in the mouse heart: βMHC, or MyH7, in embryonic cardiomyocytes, and αMHC, or MyH6, postnatally. Efforts cause hypertrophy and a shift to fetal βMHC with negative repercussion on the cardiac function.
Organ morphogenesis involves the orchestration of cell differentiation, proliferation, and migration (Fig. 6.1). During early embryogenesis, the heart is a single, relatively straight tube that bends and twists. The cardiac tube is composed of an outer layer of myocardium and an inner lining of endocardial cells, separated by an extensive extracellular matrix, the so-called cardiac jelly. Local protuberances of the cardiac jelly and associated mesenchymal cells form cardiac cushions.
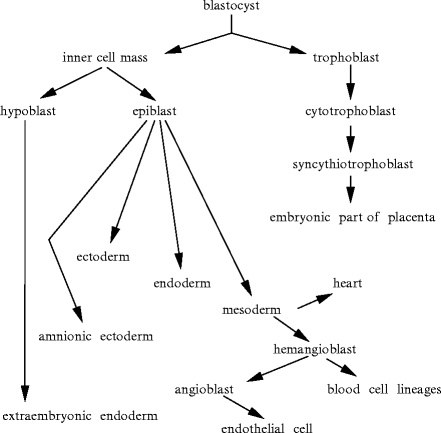
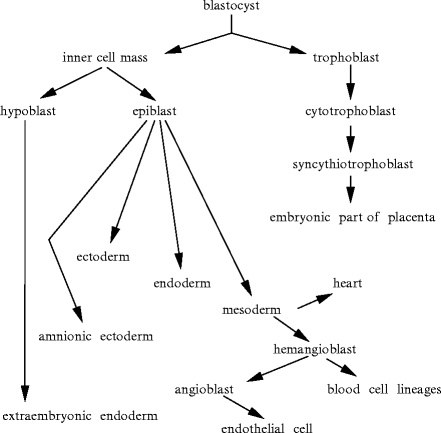
Fig. 6.1
The cardiovascular organs develop from the mesoderm. The blood vessels form independently from the heart. The heart is the first functional body’s organ.
Different myocardial progenitors form the various regions of the heart. The embryonic heart begins to pump blood before the development of valved chambers. The pumping action results from suction into the heart tube [482]. The blood stream in the developing heart favors the directed fluid motion in a stressed gel-like medium. The embryonic heart is transformed from a simple tube into a 4-chambered pump via multiple transluminal septation.
Two migratory cell populations invade the heart-forming region to regulate cardiogenesis. The cardiac neural crest originates from the neural tube. After migration into the cardiac outflow tract, it is involved in the septation of the aortic sac [483]. Epicardial cells that originate from the proepicardial serosa epithelium migrate onto the surface of the heart and create the epicardium and subepicardial connective tissue, the coronary vascular system (endothelial cells, smooth myocytes, and perivascular fibroblasts), intermyocardial and subendocardial connective tissue, as well as a small number of endocardial cells close to the sinus venosus [483].
The proepicardium gives rise to the myocardial stromal andvascular smooth muscle cells, but fewcoronary endothelial cells. Coronary vessels arise from angiogenic sprouts of the sinus venosus that returns blood to the embryonic heart [484]. Sprouting venous endothelial cells dedifferentiate as they invade the myocardium. These cells differentiate into arterial and capillary cells. Cells on the surface redifferentiate into venous cells.
After heart looping, the cardiac jelly that overlies the future atrioventricular canal and outflow tract expands into cardiac cushions. The formation of the cardiac cushions is characterized by endothelial–mesenchymal transformation of a subset of endothelial cells. During heart valve formation, a subset of endothelial cells that corresponds to future valve sites delaminates, differentiates, and migrates into the cardiac jelly [485].
6.1.1 First and Second Heart Fields
Soon after gastrulation, heart formation starts in the precardiac anterior mesoderm (first and second heart fields) under the coordinated action of inductive and repressive signals from neighboring endoderm and ectoderm (Table 6.2). On the other hand, posterior mesoderm is a blood-forming region.
Table 6.2
Examples of signaling molecules involved in cardiac specification (Source: [486]; FGF: fibroblast growth factor; GATA: DNA sequence GATA-binding transcrition factor; Myocd: myocardin; NKx2: NK2 transcription factor-related homeobox protein; SHh: sonic Hedgehog; SRF: serum response factor; TBx: T-box transcription factor; TH: tyrosine hydroxylase). Inhibitors of bone morphogenetic proteins (BMP) — noggin and chordin — secreted from the notochord limit the amount of developing cardiac tissue. They also retard the differentiation of a population of cardiac precursors that will form the outflow and inflow tracts of the mature heart. Cerberus-related protein is an extracellular, multivalent antagonist that binds to growth factors of the transforming growth factor (TGFβ) superfamily, Nodal and BMPs, as well as Wnt morphogens.
Ectoderm | Wnt1/3 |
---|---|
Endoderm | BMP2, FGF8, SHh |
(cardiogenic inducers) | Cerberus |
Mesoderm | |
Notochord | Noggin, chordin |
Cardiac mesoderm | GATA4/5/6, MyoCd, NKx2.5 SRF, TBx20, TH, Wnt11 |
Mesodermal cells that differentiate into cardiac cells during cardiogenesis segregate into 2 distinct populations of cardiac progenitors: the primary heart and anterior heart fields that contribute to the left ventricle and right ventricle and outflow tract, respectively. Unlike the first heart field, second heart field cells remain undifferentiated until they migrate into the forming heart.
Both heart field cells are tagged by the homeobox NK2 transcription factor-related locus-5 proteinNKx2-5 encoded by homeobox NKX2-5 gene,2 whereas transcription factor Islet-1 (Isl1)3 is only involved in the differentiation of second heart field cells. Second heart field cells persist in the postnatal heart. These progenitors can differentiate into several types of cardiac cells.4 In human fetal hearts at 11 and 18 weeks of gestation, Isl1 + cells lodge in the right atrium and outflow tract that are both second heart field-derived structures as well as left atrial wall and appendage [487].
6.1.2 Heart Growth Patterns
During cardiogenesis, heart chambers accommodate changes in load associated with the body’s development to fulfil increasing needs. Certain cell subpopulations participate to a greater extent to cardiogenesis characterized by marked changes in size (heart chambers having specific dimensions) and tissue architecture. Hence, cardiogenesis does not follow a gradual expansion during which all involved cardiac cell types proliferate and contribute equally to heart remodeling and reshaping.
In the zebrafish heart, expansion of the surface area results from proliferation of a subset of cells from the embryonic wall, each of which creating a muscular patch of given size and shape. During embryonic maturation, morphogenesis continues from a primitive structure. The ventricular wall thickens, not by proliferation of the juvenile wall (original inner layer), but by a new outer layer of muscle (cortical muscle) generated by some dominant clones of proliferating cells that may arise from internal trabecular muscle. In fact, cortical cardiomyocytes initially emerge from internal myofibers that breach the juvenile ventricular wall and then expand over the surface [488]. Both inner and outer layers persist in the adult heart. Therefore, 3 ventriculomyocyte lineages localize to the adult organ: primordial, trabecular, and cortical myocardial lineage, created in this order from each other [488].
6.1.3 Epithelial–Mesenchymal Transition
Epicardium, coronary beds, and conduction paths interact during cardiogenesis. Cells of the proepicardial organ (PEO), a more or less diffuse cluster of extracardiac mesothelial cells, are precursors for epicardial epithelial, myocardial connective tissue, coronary smooth muscle, and endothelial cells. After proliferation, PEO cells travel over the myocardial surface and differentiate into an epithelium to form the primitive epicardium. A set of epithelial cells migrate into the subepicardium and undergo anepithelial-to-mesenchymal transition.5
Epithelial-to-mesenchymal transformation is implicated in manifold steps of cardiovascular morphogenesis, such as heart valve development, coronary artery formation, and inflow and outflow tract septation [489]. Epithelial–mesenchymal transition is the first step in morphogenesis that transforms the mesenchyme into functional structures, such as septa and valves, required for unidirectional pumping activity.
A set of epithelial cells in the cardiac wall generate cardiac fibroblasts and participate in coronary vasculogenesis. Another set interacts with myocardioblasts for myocyte differentiation into cardiac Purkinje cells. Certain epicardium-derived cells migrate in the core of the endocardial cushions to produce atrioventricular valve leaflets. Epithelial-to-mesenchymal transformation occurs also in adult life, especially in cardiovascular diseases such as atherosclerosis, intimal hyperplasia, and aneurysms.
Ubiquitousperiostin is involved in epithelial–mesenchymal transformation and subsequent mesenchymal maturation, with differentiated mesenchyme and condensed matrix in embryonic heart valves. Periostin is also highly expressed in the myocardium in patients with heart failure. Periostin inhibits cardiomyocyte spreading and adhesion of cardiac fibroblasts [490].
During normal heart development, epicardial-derived cells (EPDC) undergo an epithelial–mesenchymal transition in response to BMP, FGF, and TGFβ released from the myocardium (Vol. 2 – Chap. 3. Growth Factors). Secreted myocardialthymosin-β4 then induces EPDC migration into the myocardium, where they respond to angio- (VEGF and FGF2) or arteriogenic (PDGF and TGFβ) growth factors and differentiate into endothelial and smooth muscle cells, respectively, leading to a capillary plexus and stabilizing coronary vessels.
6.1.4 Vasculo- and Angiogenesis
Embryonic coronary vessel development involves the activation and proliferation of epicardial cells, followed by an epithelial–mesenchymal transition. Mural and mesenchymal cell markers such as PDGFRβ are upregulated [491]. Signaling by PDGF that causes the formation of stress fibers and loss of intercellular contacts of epicardial cells is needed for epicardial cell proliferation.
Endothelium-lined tubes construct a functional circulatory network. The formation of endothelial vacuoles followed by intra- and intercellular fusion drives vascular lumen genesis [492]. These endothelial pinocytic vacuoles quickly occur, fuse together, and enlarge to create the tube lumen. Endothelial cells form extensive contacts between themselves and merge their intracellular vacuoles without cytoplasmic mixing. The vacuolar compartments in adjacent endothelial cells associate and form a continuous lumen in developing blood vessels.
During embryogenesis, the generation of new cardiomyocytes from progenitor cells and existing cardiomyocytes is associated with the development of the coronary vasculature from progenitors that originate from the endothelium as well as proepicardial organ and neural crest [493]. Coupling of myocardial and coronary development is mediated by bone morphogenetic proteins and fibroblast (FGF) and vascular endothelial (VEGFa) growth factors.
The myocardial development relies [493]: (1) in cardiomyocytes, on growth factors and their receptors, such asVEGFa,BMPR1a (ALK3),FGFR1 and FGFR2;MAPK14 (P38MAPKα), TxnRd2 thioredoxin reductase, and transcription factors bHLHa26 (or HAND2), bHLHa27 (or HAND1), GATA4, ZFPM2,6 and SRF; (2) in the proepicardial organ, on retinoid X receptor RXRα (or NR2b1) and Wilms tumor suppressor WT1; (3) in the endothelium, onephrin-B2, endothelial receptorTIE2,MAPK7 kinase,PTen phosphatase, neurofibromin NF1, andcaspase-8; and (4) in neural crest cells, on BMPR1a andPaired box Pax3 protein.
6.1.5 Neurogenesis
The autonomic nervous system involved in the body’s homeostasis and stress defense includes not only the sympathetic and parasympathetic neurons and their centers in the central nervous system, but alsoadrenal medulla. The sympatheticoadrenal lineage corresponds to a common progenitor of adrenergic sympathetic neurons and medullary cells of the adrenal gland. Progenitors of sympathetic ganglia originate from neural crest cells, which constitute an embryonic progenitor cell population emigrating from the dorsal aspect of the neural tube. Neural crest cells generate sympathetic and medullary cell lineages during embryogenesis.The dorsal aorta acts as a morphogenetic center that coordinates migration and segregation of neural crest cells [494].Bone morphogenetic proteins produced by the dorsal aorta stimulate the production ofCXCL12 chemokine andneuregulin-17 (Sect. 6.1.8.3) in the para-aortic region. These molecules are chemoattractants. Later, BMP signaling instructs sympatheticoadrenal specifications.
Cardiac sympathetic noradrenergic neurons neednerve growth factor for survival and development.Neurturin (Nrtn), a member of the family of glial cell line-derived neurotrophic factors (GDNF), is required for cardiac parasympathetic cholinergic neurons [495]. This neurotrophic factor controls neuron survival and differentiation.
However, because a certain fraction of the population of cardiac cholinergic neurons persists in the absence of neurturin, additional neurotrophic factors intervene. Members of the GDNF family target their cognate coreceptors (GDNFRα1–GDNFRα4) coupled to transmembranereceptor protein Tyr kinase Rearranged during transfection (ReT; Vol. 3 – Chap. 8. Receptor Kinases).8 The neurturin receptor, i.e., the GFRα2–ReT complex, is expressed by cardiac parasympathetic neurons. Receptor GDNFRα29 is a glycosylphosphatidylinositol (GPI)-anchored cell surface receptor for both GDNF and Nrtn that primes activation of ReT receptor. Neurturin binds preferentially to GDNFRα2 coreceptor compared to GDNFRα1.10
6.1.6 Valvulogenesis
Cardiac cushions protrude from the cardiac jelly and underlying myocardium to form thin, tapered leaflets with a single endothelial cell layer and a central matrix with collagen, elastin, and glycosaminoglycans [485]. Endothelial–mesenchymal transformation allows the generation of the endocardial cushion, the primordia of valves, and septa of the heart. The final atrioventricular valves derive from endocardial cushions in atrioventricular canal. The development of ventriculoarterial valves from the outflow tract relies on a population of neural crest cells from the branchial arches that migrates to the distal outflow tract and contributes to aortopulmonary septation. Nevertheless, resident cells of leaflets of all the cardiac valves have an endothelial origin [485].
Developingcardiac valves, like other avascular tissues, express transcription factors, such assex-determining region Y (SRY)-box Sox9,nuclear factor of activated T cells NFAT8,Runt-related transcription factor Runx2, and Msh homeobox gene product Msx2, as well as growth factors, such as BMP2 and TGFβ2.
Numerous signaling axes regulate endothelial proliferation and differentiation in developing and postnatal heart valves, such as VEGF–NFAT2, Notch–TGFβ, Wnt–β-catenin, BMP–TβR, HBEGF–HER, and NF1–Ras [485] (Table 6.3).
Table 6.3
Factors of cardiac valve development and remodeling (Source: [485]). Various signaling pathways and transcriptional regulators coordinately regulate valvulogenesis (BMP: bone morphogenetic protein; HA: hyaluronic acid; HAS: hyaluronan synthase; HBEGF: heparin-binding epidermal growth factor-like growth factor; HER: human epidermal growth factor receptor; NF1: neurofibromin-1; NFAT: nuclear factor of activated T cells; TGF: transforming growth factor; VEGF: vascular endothelial growth factor).
Factor | Effect |
---|---|
BMP2/4/6 | Epithelial–mesenchymal transition |
HA | Epithelial–mesenchymal transition, migration |
via HER2 and HER3 | |
HBEGF | Inhibition of cell proliferation |
NF1 | Inhibition of Ras GTPase (of cell proliferation) |
Notch | TGFβ signaling |
TGFβ | Cell mobilization |
VEGF | Endothelial cell proliferation via NFAT2 |
Wnt | Production of BMP4, versican, HAS2 |
The Wnt–β-catenin signaling may control production of the growth factors such as bone morphogenetic proteins as well as structural elements, such asversican and hyaluronic acid, that are needed for epithelial–mesenchymal transition [485].
Vascular endothelial growth factor controls the development of endocardial cushion and cardiac valve. Subtype VEGF165 signals via the VEGFR1–VEGFR2–neuropilin-1 complex on endothelial cells and their effectors, such as inositol triphosphate, diacylglycerol,extracellular signal-related kinases, and, in valve endothelial cells, transcriptionfactor NFAT2 (or NFATc1) [497]. Factor VEGF acts via VEGFR2 and promotes NFAT2 nuclear localization to increase the proliferation of valvular endothelial cells. In addition to Ca2 + release from its store upon IP3 stimulation, Ca2 + may enter endothelial cells in developing cardiac cushions through connexin-45-based hexameric gap junctions and activateprotein phosphatase-3 [485]. Phosphatase PP3, in turn, dephosphorylatesNFAT2 that then moves into the nucleus, where it interacts with transcriptional regulators such asActivator protein-1 to trigger gene transcription.
Activated Notch is converted from a transmembrane receptor to a transcriptional coactivator that participates in the control of epithelial–mesenchymal transition of endocardial cushions. Notch signaling increases the level oftransforming growth factor-β2. The latter increases the activity of Snail transcription factor, thereby downregulating cadherin-5 and endothelial cell separation. On the other hand, SMAD6 attenuates TGFβ signaling in developing valves [485].
The major TGFβ family members implicated in cardiogenesis include TGFβ2, TGFβ3,BMP2, and BMP4 factors. Transforming growth factor-β3 is synthesized in transforming endothelial and invading mesenchymal cells, whereas bone morphogenetic protein-2 is expressed in the myocardium. Myocardial signals upregulate the expression of endothelial TGFβ3 that acts synergistically with BMP2 in the atrioventricular canal to prime the epithelial–mesenchymal transition [498]. The production of BMP2 and BMP4 is stronger in the myocardium underlying developing atrioventricular canal and outflow tract, respectively. Isotype BMP6 is expressed in endocardium, myocardium, and mesenchyme of the developing heart, especially in the atrioventricular canal [485].
Heparin-binding epidermal growth factor-like growth factor (HBEGF) is strongly expressed in the endocardium overlying the cushion-forming area. It is cleaved from the transmembrane precursor proHBEGF byADAM17 metallopeptidase. It can bind human epidermal growth factorreceptors HER1 and HER4. It limits mesenchymal cell proliferation once bound to HER1–HER2 heterodimer [485].
On the other hand, activation of HER2–HER3 heterodimer byhyaluronic acid increases the epithelial–mesenchymal transition and migration via Ras signaling [485]. Hyaluronic acid is produced by hyaluronan synthases (HAS1–HAS3). Enzyme HAS2, the main enzyme for hyaluronic acid production during embryogenesis, is required for the formation of the cardiac jelly. This glycosaminoglycan of the extracellular space regulates ligand availability. It interacts with numerous matrix constituents such as versican, a major proteoglycan of the cardiac jelly.
Small Ras GTPase is activated by receptor protein Tyr kinases to heighten mesenchymal proliferation. It can then signal via MAPK modules.Neurofibromin-1 is a Ras-specific GTPase-activating protein (RasGAP) that inactivates Ras GTPase. Neurofibromin-1 can diminish endothelial and/or mesenchymal cell proliferation. Small Ras GTPase can also interact with NFAT2 to control gene transcription [485]. Neurofibromin-1 modulates the timing of NFAT2 intranuclear localization to avoid hyperplasia of cardiac cushions.
Transcription factor Sox9, in conjunction with Sox5 and Sox6, targets the CHM1 gene in avascular tissue that encodeschondromodulin-1.11 In the interstitial space, chondromodulin-1 inhibits endothelial cell proliferation and angiogenesis [499]. Chondromodulin-1 expression persists in normal cardiac valves throughout life. It is downregulated in degenerative cardiac valves. Loss in chondromodulin-1, indeed, leads to vascularization, calcification, lipid deposition, and inflammation of cardiac valves.
With its structural and functional heterogeneity, the heart achieves a coordinated contraction of its myofiber population in the 2 in-series pumps with its twinned chambers to eject blood in both circulations during each cycle. This composite material is optimally organized. The activation phase of the myofibers matches their mechanical heterogeneity for a suitable electromechanical coupling.
6.1.7 Postnatal Growth
During the fetal life, cardiomyocytes proliferate. Although from birth to adulthood heart mass rises, cardiomyocyte proliferation slows around birth [500]. Most of the postnatal increase in cardiomyocyte mass results from hypertrophy rather than cell division, whereas other cardiac cell types give rise to multiple new cells during postnatal growth of the heart. Nonetheless, long-lived cardiomyocytes can synthesize DNA after birth. In humans, postnatal cardiomyocytes can actually produce DNA without nuclear division (polyploid nucleus). In addition to nuclear diploidy and even sometimes polyploidy, a certain fraction (about 25%) of the cardiomyocyte population undergoes a final nuclear division without cell division. Bi-, tri-, and tetranucleated cells represent about 25.5, 0.4, and 0.1% of the cardiomyocyte population [501].
Cardiomyocytes are able to partly repopulate human myocardium, but the limited functional recovery after myocardial injury clearly demonstrates insufficient regeneration of cardiomyocytes. Cardiomyocytes renew with about 1% annual turnover at 25 years old that gradually decays down to 0.45% at 75 years old [502].
Myocardin (Myocd) is a potent transcriptional coactivator ofserum response factor expressed exclusively in cardiac and smooth myocytes during postnatal development. It regulates maintenance and adaptation of the heart, especially cardiomyocyte structure and organization of intercalated discs and sarcomeres [503]. Myocardin links to serum response factor to synergistically activate gene transcription for heart adaptive response to growth factors and hemodynamic stress.
Myocardin-regulated genes encode cardiac cytoskeletal and myofibrillar structural proteins, such as cardiac α-actin-1 (ACTC gene), α-actinin-2 (ACTN2 gene), cardiac myosin heavy chain-7 (i.e., slow isoform βMHC; MYH7 gene), desmin (DES gene), dystrophin (DMD gene), and tropomyosin-1 (tropomyosin-α chain; TPM1 gene).
Growth factors activateextracellular signal-regulated kinases ERK1 and ERK2. The latter, in turn, phosphorylate myocardin isoform-B (Ser812, Ser859, Ser866, and Thr893) [504].
The synthesis of protein Ser/Thr kinase PIM1 in the myocardium decreases during postnatal development, but re-emerges after acute injury.Cardioprotection associated with PKB activation induces PIM1 expression (downstream from PKB) to impede cardiomyocyte apoptosis and enhance calcium dynamics [505].
6.1.8 Regulators
Cardiac specification requires a permissive environment. Anterior endoderm adjacent to the heart-forming region, the anterior mesoderm, provokes and maintains cardiogenic fate. This endoderm region secretes many messengers, such as fibroblast growth factors, activins and bone morphogenetic protein BMP2 of the TGFβ superfamily, and insulin-like growth factor-2 that promote cell survival and proliferation of cardiogenic cells and differentiating myocytes [483].
Cardiogenesis relies on few recycled functional modules that integrate into protein networks according to a given spatiotemporal control to drive the stages of organ development [506]. Complicated organ are thus built using simple building blocks that coordinate morphogenesis.
6.1.8.1 Transcription Factors
Homeobox gene products, such as members of the homeobox HOX and NK2 families, as well as transcription factors of the helix–loop–helix superfamily such as the myogenic genes (e.g.,myogenin [Myog], or myogenic factor-4,myogenic differentiation factor-1 [MyoD or MyoD1],myogenic factor-5 [MyF5], andmyogenic regulatory factor MRF4) participate in cell lineage commitment.
Cardiac and endothelial cell lineages arise from a common progenitor. Synthesis of transcription factorE-twenty six (ETS)-related protein ETSRP71 is transiently induced in the endocardium and endothelium of the embryo byNKx2-5 that binds to the NKX2-5 response element of the Etsrp71 gene promoter [507]. This factor targets TIE2 receptor to specify cell fate in the developing heart.
Transcription factors, such as NKx2-5,DNA sequence GATA-binding protein GATA4, andmyocyte-specific enhancer factor-2C (MEF2c), are required for normal cardiogenesis that is regulated by signaling molecules (e.g., bone morphogenetic proteins, fibroblast growth factors, and Wnts). These signals induce or prevent the early stage of cardiomyocyte differentiation.
In vertebrates, 3 members of the GATA family of zinc finger transcription factors (GATA4–GATA6) operate in the heart in addition to products of the NK family of homeobox genes [483].Serum response factor, a member of the MADS box family, mediates the transcription launched by various extracellular stimuli. Serum response factor is inhibited by a homeodomain-only protein HOP that is unable to bind to DNA, the synthesis of which is regulated by NKx2-5.Myocardin of the family of SAP domain-containing nuclear proteins activates promoters of cardiomyocyte genes via its association with serum response factor.
High-mobility group, nuclear protein HMGA212 regulates cardiomyocyte differentiation, as it associates with BMP-responsive transcription factorsSMAD1 and SMAD4 to synergistically activate the promoter of the cardiac NKX2-5 gene [508].
Transcription factorsnuclear factor of activated T-cells NFAT3 and NFAT4 are required for normal enzymatic activity of complex-2 and -4 of the respiratory chain and mitochondrial oxidative activity [509].
Transcription factor E2F4 activates proliferation of developing cardiomyocytes during cardiogenesis.13 It operates as both a repressor and activator of cell proliferation. It accumulates in the nucleus at the end of the S phase of the cell division cycle, remains nuclear during mitosis, and disappears at the end of cytokinesis [510]. Proliferation of cardiomyocytes ceases during the first weeks after birth. In cardiomyocytes, expression of E2F4 declines during embryogenesis. Cardiomyocyte hypertrophy then occurs. Reinduction of proliferation of postnatal cardiomyocytes relies on E2F4 production.
6.1.8.2 Transcriptional Control of Chamber Formation
Two Hairy enhancer of Split (HES)-related transcription repressors — HRT1 and HRT2 — are produced in atrial and ventricular progenitor cells [483]. They are controlled by the Notch signaling pathway.
Iroquois-related homeobox protein Irx4 labels ventricular progenitor cells in the cardiac crescent. Once chambers are formed, Irx4 is exclusively expressed in ventricles [483].
The transcription factors, heart and neural crest derivatives expressed proteinsHAND1 and HAND2 (or bHLHa27 and bHLHa26, respectively) are also involved in ventricle formation. Both factors are coexpressed in the primary heart tube. Afterward, bHLHa26 and bHLHa27 accumulate in the right and left ventricle, respectively [483].
Myocyte enhancer factor-2C, which is phosphorylated byP38MAPKα andERK4 (or ERK5) kinases, is needed for right ventricle construction.
On the other hand, the atrial myocardium is stamped byGATA4 andTBx5 factors.14 Nuclear receptor NR2f1 is restricted to the inflow tract myocardium [483].
The allocation of cells to the atrial and ventricular lineages is also regulated byretinoic acid. Retinoic acid-synthesizing enzyme retinaldehyde dehydrogenase RAlDH2 is specifically synthesized in the posterior heart region, where retinoic acid participates in the formation of atria [483].
6.1.8.3 Tyrosine Hydroxylase
Tyrosine hydroxylase, ot tyrosine 3-monooxygenase, the rate-limiting enzyme in catecholamine synthesis, is strongly produced in the cardiac mesoderm. Tyrosine hydroxylase catalyzes the conversion of Ltyrosine to L(3,4)-dihydroxyphenylalanine (LDOPA)16 that generates dopamine, a catecholamine neurotransmitter and hormone, by aromatic amino acid decarboxylase.
Catecholamines present during cardiogenesis comprise LDOPA (predominantly) and dopamine. Molecule LDOPA enhances BMP2 production. It may be an important modulator of cardiac pacemaker activity, as it leads cardiac precursor cells to a sinoatrial fate [511].
Tyrosine hydroxylase regionalizes the production of atrial myosin heavy chain (aMHC1) and TBx5 factor to the posterior region of the heart tube during initial cardiomyocyte differentiation [511].Retinoic acid synthesis is required for Tyr hydroxylase activity, hence expression of aMHC1 and TBx5 proteins.
6.1.8.4 Growth Factors
6.1.8.5 FGF
6.1.8.6 Neuregulin-1
Signaling triggered byneuregulin-1, a member of the epidermal growth factor (EGF) family (Sect. 6.1.5), and its heterodimericreceptor protein Tyr kinase HER2–HER4 participates in cardiogenesis as well as proper structure and function of the adult heart.17
Cardiomyocytes in the adult myocardium produce HER2 and HER4 receptor protein Tyr kinases. Neuregulin-1 binds to HER4 with high affinity; HER4 dimerizes preferentially with HER2 receptor.18 Receptor HER2 of the HER2–HER4 heterodimer acts as a non-autonomous amplifier. In the heart, neuregulin-1 primes [514]: (1) the Src–FAK, (2) SHCa–MAPK, and (3) PI3K–PKB pathway.
Neuregulin-1 contributes to cardiomyocyte differentiation from stem and progenitor cells, migration, and proliferation during cardiogenesis as well as during the postnatal myocardial development that involves the population of mononucleated cardiomyocytes and cardiac regeneration in damaged adult hearts [514].19
6.1.8.7 TGFβ
Transforming growth factor-βreceptor TβR3, a coreceptor for members of the TGFβ superfamily, resides in the heart fields [483]. It acts as a permissive factor in cardiogenesis. Coreceptor TGFBR3 is involved inNodal signal transduction. Nodal signaling contributes to endoderm formation [483].
Member of the tumor-necrosis factor superfamily TNFSF12 regulates proliferation of several cell types, among which are cardiomyocytes. This type-2 transmembrane glycoprotein is processed into a soluble cytokine. In neonatal cardiomyocytes, TNFSF12 upregulates the expression of activators of cardiomyocyte proliferation, such as nuclear marker of cellular proliferation Ki67, mitotic marker phosphohistone-3 (H3P),cyclin-D2, andAurora-B kinase, as well as represses that ofcyclin-dependent kinase inhibitor CKI1b [516]. Cytokine TNFSF12 targets TNFRSF12a receptor that signals via activatedextracellular signal-regulated kinases andphosphatidylinositol 3-kinase as well as inhibition ofglycogen synthase kinase-3β. In adult cardiomyocytes, induction of cell cycle re-entry requires TNFRSF12a re-expression.
6.1.8.8 Morphogens
The NKX2-5 promoter contains several SMAD binding sites. Bone morphogenetic protein BMP2 can elicit the expression of myocardial lineage markers. On the other hand, noggin that binds to and inhibits BMPs (as it precludes TGFβ family ligands from binding to their receptors) prevents myocardial differentiation. In addition, several members of the BMP family with overlapping functions are expressed in the cardiac mesoderm, such as BMP5 and BMP7 [483].
6.1.8.9 Hedgehog
Both sonic and indian Hedgehog are expressed in the pharyngeal endoderm during cardiac crescent formation [483]. They operate as permissive rather than inductive factors via Smoothened receptor during cardiogenesis.
6.1.8.10 Wnt
Wnt morphogens operate as positive and negative regulators of the primary heart field. Subtype Wnt1 in the heart field blocks myocardial differentiation and induces blood formation. Canonical Wnt signals that operate via β-catenin, such as Wnt2a, Wnt2b, Wnt3, and Wnt8 regulate specification of cardiac cell types from the early mesoderm [517]. On the other hand, Wnt5a and Wnt11 trigger the non-canonical Wnt pathways during cardiogenesis. Subtype Wnt11 activates, via G protein,PKC,JNK, andCamK2 kinases.
An alternative branch of the Wnt pathway uses Jun N-terminal kinases for gastrulation movements.Diversin20 interacts with 2 components of the canonical Wnt pathway,casein kinase-1ε, and axin. It suppresses Wnt signals mediated by the canonical β-catenin pathway and stimulates signaling via Jun N-terminal kinases [518]. Diversin controls fusion of heart precursors [519]. Diversin binds Disheveled to activate Rac and Rho GTPases and then Jun N-terminal kinase.
6.1.8.11 Chromatin Remodelers
The large ATP-dependent chromatin-remodeling switching/sucrose non-fermenting (Swi/SNF)-like BAF (Brahma-related gene [BRG1]/Brahma [Brm]-associated factor) complex uses the energy of ATP hydrolysis to modify the chromatin structure made of DNA and histones, thereby activating or repressing gene expression. The chromatin-remodeling Swi/SNF-like BAF complex that is involved in cardiogenesis is characterized by a combinatorial assembly of subunits that produces distinct functional complexes.
In particular, the cardiogenic BAF complex comprise Swi/SNF-related, matrix-associated, actin-dependent regulators of chromatin SMARCd1 to SMARCd3 subunits.22 Cardiac-enriched BAF complex subunit SMARCd3 cooperates with GATA4 to provoke NKx2-5 production. Moreover, SMARCd3 and GATA4 cooperate with TBx5 cardiogenic transcription factor to activate genes that encode regulators of cardiogenesis, such as MEF2, NKx2-5, HAND, and Isl1 [522]. Factors SMARCd3, GATA4, and TBx5 promote differentiation into contracting cardiomyocytes and repress non-cardiac mesodermal genes.
Chromatin-remodeler and transcriptional activator SMARCa423 participates in the regulation of gene expression during cardiac growth and differentiation. The SMARCa4-containing BAF complex comprises 12 subunits. In embryos, SMARCa4 promotes myocyte proliferation, as it maintains expression of BMP10 and suppresses that of cyclin-dependent kinase inhibitor CKI1c [523]. It interacts withhistone deacetylase and polyADPribose polymerase to repress αMHC (MyH6) and activate βMHC (MyH7) isoform. The chromatin-modifying HDAC–PARP–BAF complex regulates cardiac hypertrophy. It assembles on MHC promoters, where it can interact with transcription factors such as thyroid hormone receptors TRα1 and TRβ1 (a.k.a. nuclear receptors NR1a1 and NR1a2), transcriptional enhancerTEA domain-containing family member TEAD1,24myocyte enhancer factor MEF2,serum response factor (SRF),GATA4, andNFAT to control MHC expression [523]. In adults, SMARCa4 is silenced in cardiomyocytes. It is reactivated by cardiac stresses and forms a complex with its embryonic partners HDAC and PARP1 to induce a pathological MyH6-to-MyH7 shift.
Developing myocardial cells respond to signals from the endocardial layer to form a network of trabeculae that characterize the ventricles of vertebrate hearts. Trabeculation requires the chromatin remodeler SMARCa4 to repressADAM metallopeptidase with thrombospondin-1 motif ADAMTS1 produced in and secreted by the endocardium that overlies developing trabeculae to achieve an extracellular environment in the cardiac jelly that supports trabecular growth [524]. Later, ADAMTS1 degrades the cardiac jelly and prevents excessive trabeculation.
6.1.8.12 Matrix Proteoglycans
Perlecan is a heparan sulfate proteoglycan in basementmembranes that binds to integrins and dystroglycan, interacts with extracellular matrix components, and recruits growth factors and modulates their activity. Perlecan stabilizes the heart wall during cardiogenesis as well as in adults after injury. Basement membranes that lack perlecan deteriorate and lead to cell detachment in the ventricle and outflow tract and blood leakage into the pericardial cavity [525].
At the onset of cardiogenesis, perlecan serves as an adhesive substrate for cardiomyocytes with laminin and collagen-4 and maintains mechanically stressed basement membrane surrounding cardiomyocytes to ensure mechanical stability. At later stages, heart stability is achieved by formation and maturation of intercellular contacts such asintercalated discs composed of desmosomes and adherens junctions that are composed of γ-catenin25 and cadherin, respectively.
6.1.8.13 MicroRNAs
Cardiogenesis depends on the spatiotemporal expression of microRNAs.MicroRNAs control the expression of messenger RNAs that are translated into regulators of cardiogenesis, propagation of electrochemical waves, and myocardium hypertrophy and contractility (Table 6.4).
Table 6.4
MicroRNAs and heart structure and activity in mice (Sources: [526–530]). MicroRNAs regulate cardiogenesis and growth, conduction of the electrochemical signal, and myocardium contraction. They include muscle-specific microRNAs (miR1, miR133, and miR208) and other microRNAs (e.g., miR18b, miR21, and miR195). Cardiac miR1 targets a gap junction protein and cardiac transcription factor Irx5 that regulates the nodal tissue. Overexpression of miR1 is associated with cardiac arrhythmia. Loss in miR1-2 leads to ventricular septum defects (septal holes), cardiomyocyte hyperplasia, and abnormal conduction in the ventricular nodal tissue. MicroRNA-133 prevents cardiac hypertrophy in response to mechanical and chemical stimuli. Heart-specific miR208 is transcribed from a sequence of the intron of the gene of the heavy chain of α-myosin. MicroRNA-208 is required for the expression of β-myosin heavy chain during stress or hypothyroidism. Hyperpolarization-activated and cyclic nucleotide-gated channels (HCN) are responsible for depolarizing pacemaker “funny” current i f.
Type | Cardiac effect |
---|---|
MicroRNA-1 | Growth, conduction |
(GJA1 [Cx43] and KCNJ2 [KIR2.1]), | |
automaticity (HCN2 and HCN4 [i f]) | |
MicroRNA-1-2 | Morphogenesis, CMC number, conduction |
MicroRNA-133 | Growth, repolarization |
(KCNQ1 [KV7.1] and KCNE1 [minK; i K,s]) | |
(KCNH2 [KV11.1; i K,r]), | |
automaticity (HCN2 [i f]) | |
MicroRNA-208 | Growth |
MicroRNAs commonly bind to mRNAs and inhibit mRNA translation with or without mRNA cleavage. The most abundant microRNAs in the myocardium are miR1, miR133, miR126-3p, miR30c, miR26a, and the (lethal) Let7 class [526]. MicroRNAs MiR1, miR133, and miR208 are specific to striated myocytes. The microRNA-1 set comprises 2 transcripts miR1-1 and miR1-2 that are encoded by distinct genes. The miR133 set includes miR133a-1, miR133a-2, and miR133b. MicroRNA-208 is encoded by an intron in the MYH6 (αMHC) gene.
Muscular microRNAs, such as miR1 and miR133, are mainly controlled by myogenic transcription factors. MicroRNA-1 targets histone deacetylase HDAC4, a transcriptional repressor of muscle gene expression. It also controls cardiogenesis in mice, as it regulates cardiac transcription factor bHLHa26 [531]. MicroRNA-133 enhances myoblast proliferation by repressing serum response factor that acts in sarcomere organization.
Serum response factor is controlled by other regulators, such as GATA4, NKx2-5, and myocardin, as well as microRNAs, such as miR1-1, miR1-2, miR21, miR133, miR206, miR214 [531]. Conversely, serum response factor regulates microRNAs such as miR1-1, miR1-2, miR133, and miR129 in cardiomyocytes.26
MicroRNA-138 is required to establish distinct identity of cardiac structures and appropriate chamber-specific gene expression patterns during cardiogenesis [532]. MicroRNA-138 represses transcripts specific to atrioventricular canal in the developing ventricle.
Action of miR1 is dose sensitive [528]. MicroRNA-1-2 regulates not only cardiogenesis, but also the cardiomyocyte cycle. In mouse embryos, homozygous deletion of muscle-specific miR1-2 induces ventricular septal defects, possibly due to an increased level of bHLHa26 transcription factor or HAND2 regulator). Surviving mice exhibit cardiac arrhythmias caused by defects in potassium channels resulting from increased abundance of the transcription factor Irx5, a repressor of the KCND2 gene that encodesKV4.2 channel. Moreover, they have an increased rate of cardiomyocyte mitosis.
Muscle-specific miR1 and miR133 hinder myocardium growth. MicroRNA-133 targets transcripts ofRhoA andCDC42 GTPases that regulate cytoskeletal dynamics during hypertrophy, as well as nuclear factor Negative elongation factor-A (NElFa)27 that is involved in cardiogenesis [529].
Cardiac contractility depends on the expression of 2 genes that encode myosin heavy chain. Isoforms α and βMHC are contractile proteins of cardiomyocytes that are regulated in an antithetical manner by various signals. The slower adenosine triphosphatase βMHC is the dominant isoform expressed in embryonic cardiomyocytes, whereas faster ATPase αMHC is upregulated in postnatal cardiomyocytes.28
Thyroid hormone T3 stimulates αMHC and inhibits βMHC transcription after birth. Cardiac-specific miR208, encoded by an intron of the αMhc gene, controls the expression of MYH7 gene that is elevated by physical stress and hypothyroidism [530]. MicroRNA-208 targets thyroid hormone receptor-associated protein THRAP129 a regulator of the thyroid hormone receptor that hampers βMHC expression.
During cardiachypertrophy, miR23a, miR23b, miR24, miR195, miR199a, and miR214 are upregulated, whereas miR150 and miR181b are downregulated [531]. Infailing heart,30 miR21, miR29b, miR129, miR210, miR211, miR212, and miR423 are overexpressed, whereas miR30, miR182, and miR526 are underexpressed. The miR1 level increases during cardiac infarctions specifically in ischemic regions [527]. Overexpression of miR1 increases the occurrence of postinfarct arrhythmias and promotes arrhythmias in healthy hearts. Overexpression of miR1 slows conduction and depolarizes the plasma membrane, as it represses transcript of the KCNJ2 gene that encodes theKIR2.1 subunit of K + channel, which sets the resting membrane potential, and of GJA1, which encodesconnexin-43 of gap junctions.
Many microRNAs that are mainly produced by cardiac non-myocytes participate in wall remodeling. In a mouse model of cardiac failure, miR21 is upregulated and decreases the percentage of apoptoticfibroblasts, thereby favoring cardiacfibrosis. MicroRNA-21 has an anti-apoptotic effect that results from activation ofextracellular signal-regulated kinase following repression of an inhibitor of the Ras–ERK pathway, sprouty homolog Spry1 [533]. Expression of Spry1 is precluded in failing human myocardium. Subsequently, enhanced activity of the ERK pathway promotes fibroblast survival and growth factor secretion that can cause interstitial fibrosis and cardiomyocyte hypertrophy due to growth factors released from fibroblasts. In addition, miR21 is upregulated in cardiac fibroblasts after myocardial ischemia–reperfusion injury [534]. MicroRNA-21 repressesPTen phosphatase that precludes PI3K–PKB signaling and MMP2 expression in cardiac fibroblasts.
6.1.8.14 RNA-Binding Proteins
Cytoplasmic and nuclear RNA-binding proteins (RBP) bind to either double- (dsRNA) or single-stranded (ssRNA) RNAs using RNA recognition motif (RRM),31 type-1 and -2 K homology (KH) domains, zinc finger region, RGG (Arg–Gly–Gly) box, DEAD/DEAH box (associated with RNA helicase activity), Pumilio/FBF (PUF) domain, double-stranded RNA-binding motif (DSRBD), Piwi/Argonaute/Zwille (PAZ) sequence (associated with binding to short single-stranded RNAs such as microRNAs),32 Sm region (associated with small nuclear snRNA binding), cold-shock domain (CSD; involved in transcriptional and post-transcriptional regulation), among others [535, 536]. RNA-binding proteins can link to RNAs via RNA bases, ribose, and phosphate groups.
Many RBPs contain 1 or more copies of a given RNA-binding domain and auxiliary domains, whereas others have 2 or more different domains. Interdomain linkers facilitate the recognition of substrates [535]. RNA-binding modules can be combined with catalytic domains to regulate the activity of the enzyme. Many RBPs have modular structures, various modules defining the structural unit; they are composed of multiple repeats of a small number of basic domains arranged in different manners that create both RNA-binding and functional diversity [535]. Combination of a given RNA-binding domain with distinct auxiliary functional motifs adds further diversity. In addition, RBPs undergo post-translational modifications, such as phosphorylation, methylation, and sumoylation, which control RNA binding as well as their function and localization [536]. Moreover, RNA-binding proteins can dimerize [535].
Ribonucleoprotein complexes (RNP) result from association of RNAs with RNA-binding proteins that have different RNA-sequence specificities and affinities. In vertebrates, cells encode hundreds to thousands of RBPs, each with unique RNA-binding activity and between-protein interaction characteristics. A relatively small number of RNA-binding scaffolds can be involved in RNA processing.
RNA-binding proteins influence the structure and interactions of RNAs. RNA-binding proteins can be classified according to target RNA types, such as messenger RNA (mRNA), ribosomal RNA (rRNA), or transfer RNA (tRNA).
Heterogeneous ribonucleoproteins (hnRNP), or pre-mRNPs, andmessenger ribonucleoproteins (mRNP) are connected to pre-mRNAs (originally called hnRNAs) and mRNAs, respectively. Several hnRNPs shuttle between the nucleus and cytoplasm, whereas others localize only in the nucleus. They operate in transcription, stability, function, packaging (i.e., preparing pre-mRNAs for post-transcriptional processes), transport, localization, processing, and turnover, hence controlling many post-transcriptional steps in gene expression. In particular, they regulate pre-mRNA splicing, editing,33 and polyadenylation.34 Heterogeneous ribonucleoproteins bind to different sets of nascent transcripts (pre-mRNAs) at distinct times during RNA processing.35
Therefore, as RBPs are involved in the transcriptional and post-transcriptional control of RNAs, they regulate gene expression patterns during the body’s development and remodeling from embryogenesis to adulthood. RNA-binding proteins also participate in the maintenance of the stem cell state and cell-fate specification.
The signal transduction and activation of RNA (STAR) set of RBPs can be subdivided into 3 categories: SAM68, SF1, and Quaking-related (QR) subsets. Members of the STAR set function in pre-mRNA splicing, export, stability, and translation. Quaking proteins recognizes and binds to a specific sequence in the 3′UTR of target mRNAs. They control muscle development via the Hedgehog pathway [538].
Heterogeneous ribonucleoprotein Embryonic lethal, abnormal vision protein-like molecule ELAV1, or human antigen-R (HuR), selects mRNAs to be exported to the cytosol for translation, hence contributing to the regulation of myoblast differentiation [539].36 The RNA-binding protein Hermes (portmanteau for heart, RNA recognition motif (RRM)-expressed sequence) connects to mature RNAs and represses cardiogenesis [540].
The assembly of sarcomeres requires proper amounts of multiple types of proteins at a given time in a coordinated manner. Contractile proteins assemble into actin-containing thin filaments and myosin-containing thick filaments. Non-contractile proteins anchor these filaments to Z discs at sarcomere ends. The Z disc is a nodal point for signaling, in addition to its structural role in the maintenance of sarcomeres. The thin filament is also composed of tropomyosin and troponin.
Alternative splicing is involved in cardiac adaptation. Isoform switch of sarcomeric titin participate in adjustment to ventricular filling. RNA-binding motif-containing protein RBM20 regulates titin splicing [541]. Abnormal mRNA splicing that results from mutations in the Rbm20 gene produces pathological titin isoform and heart failure.
The RNA-binding protein, RBM24,37 contributes to the regulation of cardiac gene expression and sarcomeric assembly, hence cardiac contractility [542]. It controls the expression of several genes that encode constituents and regulators of the Z disc. Tissue-specific protein RBM24 is upregulated during the differentiation of human embryonic stem cells into cardiomyocytes. Agent RBM24 also intervenes in skeletal muscle differentiation via the myogenic regulatory factor, myogenin. Whereas the relatively small set of myogenic regulatory factors, such as MyoD and myogenin, governs skeletal muscle differentiation, the myocarde development is controlled by numerous transcription factors. In addition, RBM24 is also involved in vasculo- and angiogenesis.
6.1.9 Cardiac Progenitor Cells and Precursors
Cardiomyocytes come from the primary and secondary heart fields in the embryo [543]. Multipotent progenitor cells generate the 4 major cell types of the heart: cardiomyocytes as well as nodal,smooth muscle, andendothelial cells.
Cardiac progenitors express the transcription factorsNKx2-5 andinsulin gene enhancer LIM homeobox protein Islet-1 (Isl1). Factor NKx2.5 is activated in multipotent cardiac progenitor cells of early embryos. NKx2.5 + cells and their progeny populate the precardiac mesoderm located dorsal to the cardiac region and developing heart tube. Factor Isl1 is expressed transiently in multipotent cardiac progenitor cells before their differentiation. Very-low-frequency electromagnetic field raises metabolic activity in cardiac stem cells in vitro [544].
Distinct cardiac lineages, such as first and Isl1-expressing second lineage, exist according to timing of entry into the heart and differentiation. Second heart field progenitors generate cardiomyocytes that reside in the outflow tract, ventricles, and atria. Proepicardium and epicardium are sources of coronary progenitors and cardiac fibroblasts.38
Both embryonic and postnatal cardiovascular progenitor cells characterized by the expression of the transcription factor Islet-1 can differentiate into cardiac smooth muscle or endothelial cells. The adult heart contains a pool of resident SCFR + pluripotent cardiovascular progenitor cells for its regeneration [556].39
Tripotent progenitor cells from the secondary heart fields express transcription factors Islet-1 and NKx2-5 as well as vascular endothelialgrowth factor receptor VEGFR2 [545]. Certain cardiac tripotent progenitor cells express the marker brachyury at a specific stage of embryogenesis [546]. SCFR + , NKx2-5 + , differentiated embryonic stem cells that serve as cardiac progenitors create cardiac and smooth myocytes, but not endothelial cells [547]. Factor Isl1 is required for differentiation of multipotent cardiac progenitor cells into cardiac and smooth myocytes, but not endothelial cells [548].
Human pluripotent stem cells, such as embryonic and induced pluripotent stem cells generate cardiomyocytes. Cardiomyocyte specification requiresWnt signaling [549]. However, cardiomyocyte differentiation is sensitive to the timing and dose of supplied Wnt.
Vascular endothelial growth factor is one of the most important angiogenic growth factors. It has pleiotropic activities, as it influences non-endothelial cell types derived from all 3 embryonic lineages, such as pneumocytes, renal podocytes, cardiomyocytes, various neuron types, hematopoietic and cardiac stem cells, among others. The Wnt pathway triggers production of various developmental messengers, such as Nodal, BMP2 and BMP4, Noggin, Wnt3a, an Wnt8a, as well as transcription factors involved in cardiomyocyte differentiation, such as NKx2-5, TBx5, and MEF2c factors, as well as insulin gene enhancer protein Isl LIM domain-containing protein IsL1, a marker for cardiac progenitors of the secondary heart field, and Mix paired-like MixL1 homeodomain-containing protein.
Cardiac implantation of multipotent mesenchymal stem cells that express VEGF stimulates revascularization, thereby reducing infarct size. These stem cells secrete numerous paracrine factors, such as VEGF, FGF2, IGF1, HGF, and CXCL12 chemokine. They can differentiate into cardiomyocytes and endothelial cells. Their liberated paracrine factors support survival of implanted mesenchymal stem cells as well as mobilization and migration of stem cells. VEGF + mesenchymal stem cells are characterized by PKB activation that enables mesenchymal stem cell engraftment in the ischemic heart and cardiac stem cell migration [550]. Moreover, VEGF + mesenchymal stem cells recruit resident, adult, cardiac stem cells to ischemic regions. Factor VEGF fosters cell migration via VEGFR1 and VEGFR3, but not VEGFR2, although cardiac stem cells synthesize the 3 receptor types. In addition, VEGF recruits CXCR4 + cardiac stem cells via CXCL12 that targets its CXCR4 receptor [550].
6.1.9.1 Resident Coronary Vascular Progenitors
VEGFR2 + , SCFR + , resident coronary progenitor cells reside in vascular niches inside the human myocardium [551]. Vascular progenitor cells are located in the proepicardium, from whence they migrate into the myocardium. They are connected by gap junctions to vascularendothelial andsmooth muscle cells as well asfibroblasts. These self-renewing cells differentiate predominantly into endothelial and smooth muscle cells and partly into cardiomyocytes.
On the other hand, myocyte progenitor cells distributed in the cardiac crescent and pharyngeal mesoderm differentiate into cardiomyocytes to progressively constitute, together with coronary vessels, the 4-chambered heart [551].
6.1.9.2 Notch and Wnt Morphogens
Niche-resident cardiac progenitors and supporting cells expressNotch-1 receptors and Notch ligand Jagged-1, respectively. The intracellular domain of Notch-1 (NotchICD) translocates to the nucleus and activates the NKX2-5 gene [552]. NotchICD connects to RBPJκ that binds to NKX2-5 promoter, hence initiating transcription and cardiomyocyte differentiation, whereas Jagged-1 activation of the Notch1 pathway downregulates transcription factors of vascular cells.
During early stage of development, when the transcription factor NKx2-5 is not expressed, the Notch pathway mediates a coordinated regulation betweenbone morphogenetic proteins, such as BMP2, BMP6, and BMP7, that are required for cardiogenesis, andWnt pathways.40 This action can generate cardiomyocytes from VEGFR2 + hemangioblasts rather than cells of the hematopoietic, vascular endothelial, or smooth muscle lineages [553].
Wnt signaling regulates NKx2-5 + , Isl1 + multipotent cardiac progenitor cells. The Wnt–β-catenin signaling promotes expansion of multipotent cardiac progenitor cells. Notch-1 impedes accumulation of phosphorylated β-catenins in these progenitors [548]. Notch-1 and β-catenin regulate positively and negatively, respectively, the expression of cardiac transcription factors Isl1,myocardin, and SET and MYND domain-containing protein SMYD1.
During later stages of cardiogenesis, Notch-4 inhibits the maturation of the cardiac lineage. Active Notch-1 receptor in embryonic stem cells reduces their cardiac potential at late differentiation stage. Notch-4 receptor, the expression of which is restricted to the heart endothelium, promotes cardiac development from cardiac mesoderm and is able to respecify VEGFR2 + hemangioblasts to a cardiac cell fate by activating BMP signaling and inhibiting the Wnt–β-catenin pathway.
Certain cardiomyocyte progenitors derive from proepicardial cells that express T-box TBx18 transcription factor. These progenitors migrate onto the outer cardiac surface to form the epicardium, and then contribute to cardiomyocyte generation in the ventricular septum and atrial and ventricular walls [554]. However, adult TBx18-expressing epicardial cells do not yield cardiomyocytes. TBx18 + cardiac progenitors also create to cardiac fibroblasts and coronary smooth myocytes. First cells from the proepicardium–epicardium to enter the heart give rise to cardiomyocytes, whereas subsequent TBx18 + epicardial lineages generate vascular support cells and fibroblasts.
Growth factors of cardiac myogenesis include members of the bone morphogenetic protein and Wnt families. Both canonical and non-canonical Wnt pathways can promote cardiomyocyte formation. Transcription factor Sox17 acts on transcription factors of cardiac specification in primitive mesoderm, mesoderm posterior homologs MesP1 and MesP2 [560]. Transcription factor MesP1 is specifically synthesized in almost all cardiovascular precursors and considered as the earliest marker of the cardiovascular lineage. Subtype MesP1 targets Dkk1 that impedes Wnt signaling during cardiovasculogenesis [561].
Wnt signaling can act as an activator of cardiogenesis in a cell-autonomous manner (i.e., within the mesoderm) after initial cardiac commitment during discrete periods of development, although it can also inhibit early cardiac engagement in a non-cell-autonomous fashion, likely by acting on adjacent endoderm. Canonical Wnt–β-catenin pathway is required for development and differentiation of cardiac progenitors of the second heart field [562]. Wnt signaling upregulates cyclin-D2.
In addition, the Wnt–β-catenin signaling exhibits developmental stage-specific effects not only on cardiomyogenesis, but also on hematopoiesis and vasculogenesis [563]. In the early phase during embryoid body formation, it enhances differentiation of embryonic stem cells into cardiomyocytes and suppresses differentiation into hematopoietic and vascular cell lineages. In the late phase after embryoid body formation, it prevents bone morphogenetic protein signaling, thus inhibiting differentiation into cardiomyocytes and enhancing differentiation into hematopoietic and vascular cell lineages.
Coronary progenitor cells of the proepicardium at the septum transversum near the venous pole of the heart are responsible for coronary arteriogenesis (but not formation of the venous system and microvasculature) [564]. β-Catenins do not influence the formation of the proepicardium, migration of proepicardial cells to the heart, and formation of the primitive epicardium, but participate in the expansion of the subepicardial space and differentiation of epicardium-derived mesenchymal cells into coronary smooth myocytes.
6.1.9.3 Transcription Factor WT1
Another cardiomyocyte precursor of the epicardium expresses transcription factor Wilms tumor protein WT1 [555].41 WT1 + proepicardial cells arise from progenitors that express GATA4, Isl1, and NKx2-5. WT1-derived cardiomyocytes are located in the myocardium of all cardiac chambers and interventricular septum (7–10% and 18% of ventricular and atrial cardiomyocytes, respectively). They are coupled to non-WT1-derived cardiomyocytes.
6.1.9.4 ZFPM2 (FOG2) andThymosin-β4
Regulators of coronary vasculogenesis are also involved in repair of ischemic heart damage resulting from vascular insufficiency. Coronary vasculogenesis from epicardium involves ZFPM2, or Friend of GATA2 (FOG2) and VEGF [558].42
Thymosin-β4, a Gactin monomer-binding protein, promotes vascularization from the embryonic epicardium. Thymosin-β4 stimulates TIE2 + progenitor cells derived from quiescent adult epicardial cells and triggers their migration and differentiation into fibroblasts and smooth myocytes when stimulated by PDGF and TGFβ, and endothelial cells, in response to a combination of VEGF and FGF2 [559]. Thymosin-β4 product of peptidase cleavage, the pro-angiogenic Nacetyl seryl-aspartyl-lysyl-proline (AcSDKP), stimulates differentiation from adult epicardial cells into endothelial cells.
6.1.9.5 Cysteine-Rich LIM-Only Proteins
Smooth muscle α-actin exists in cardiomyocyte progenitors. During cardiomyocyte differentiation, α-actin gene activity is downregulated as soon as rhythmic contractility starts. LIM-only proteins of the CRP family are also called cysteine and glycine-rich proteins. They support the organization of proteic complexes both in the cytoplasm and in the nucleus.
Cysteine-rich LIM-only proteins CRP1 and CRP2 are expressed during the development of the cardiovascular apparatus. They bridgeGATA transcription factor toserum response factor. The SRF–CRP–GATA complex strongly activates smooth muscle genes. Together with SRF and GATA proteins, CRP1 and CRP2 facilitate smooth muscle differentiation. They are able to convert pluripotent fibroblasts into smooth myocytes. On the other hand, muscle (cardiac) LIM protein CRP3 impedes this conversion.
The level of CRP2 protein is correlated with smooth muscle gene activity [565]. Agent CRP2 is a transcription coadaptor that recruits serum response factor and remodels silent chromatin in adult cardiomyocytes toward smooth muscle gene activity.
6.2 Wall Structure
The heart wall is composed of 3 layers: the internal thinendocardium, the thick muscularmyocardium, and the external thinepicardium. Cardiac muscle is an involuntary striated muscle. The heart is enclosed in a double-wall sac, the pericardium.
The double-layeredpericardium is constituted of the outer parietal pericardium and the inner visceral pericardium, or epicardium, which is thus attached to the myocardium. The pericardial cavity separates the 2 pericardium layers. It contains a lubrificating fluid (V ∼ 25–35 ml). The parietal pericardium consists of an outer layer of thick, fibrous connective tissue and an inner serous layer with a mesothelium and connective tissue. The anchoring parietal pericardium is attached to the diaphragm and fuses with the outer wall of blood vessels entering and leaving the heart. The visceral pericardium has an external layer of flat mesothelial cells lying on a support tissue that contains elastic fibers. The coronary arteries reside in the epicardium and send branches into the myocardium.
Heart cellular population includes cardiomyocytes (at least two-thirds of all cardiac cells), nodal cells,endothelial andvascular smooth muscle cells, andfibroblasts. These various cell types are embedded in the collagen-rich extracellular matrix.
Collagen forms diverse connected structures, such as cables (collagen fibers), struts (larger crosslinked collagen bundles), nets (porous sheets of collagens), and gels (filamentous collagens associated with glycosaminoglycans). These structures bind cardiomyocytes viaintegrins (Vol. 1 – Chap. 7. Plasma Membrane) that interact with the cytoskeleton. In fact many components of the extracellular matrix bind to plasmalemmal integrins (collagen-1 to α3β1-, fibronectin to α3β1– and α5β1-, and laminin to α1β1-, α3β1-, and α7β1-integrins).
Integrins are constituents of adhesomes and signalosomes, particularly inmechanotransduction and electromechanical coupling, as integrins colocalize with gap junction proteins. Moreover, connections between integrins and the cytoskeleton regulate the functioning ofmechanosensitive ion channels. The cytoskeleton can regulate the activity of channels implicated in cardiomyocyte depolarization and subsequent repolarization [566].
Collagenous connections between adjacent cardiomyocytes register sarcomere landmarks to ensure synchronous contraction and relaxation. Furthermore, the collagen network maintains gap junctions between contiguous cardiomyocytes as well as between cardiomyocytes and nodal cells to favor the electrical connectivity.
Thecollagen turnover is regulated by: (1) stimulators, such asangiotensin-2,43endothelin-1,catecholamines,aldosterone,fibroblast growth factor FGF2,insulin-like growth factor, among others, as well as (2) inhibitors, such asprostaglandins,nitric oxide,natriuretic peptides, etc. [567].
Themyocardium contains mainly cardiomyocytes. In both ventricles, papillary muscles and chordae tendinae tether the valve. The extracellular matrix couples the different cardiac cell types and conveys chemical, mechanical, and physical signals that regulate cell functioning.
Theendocardium is composed of 3 layers. The outer layer is made of collagen fibers, which merge with collagen surrounding adjacent cardiomyocytes. This layer contains the Purkinje fibers (cardiac Purkinje cells) of thenodal tissue. The thickest middle endocardial layer contains more regularly arranged collagen fibers and elastic fibers arranged in parallel. The inner layer is constituted of endothelial cells (EC), which are continuous with the vessel endothelium. The endocardium is thicker in the atria than in the ventricles.
The heart has a fibrous skeleton with its central fibrous body, which prevents early propagation of action potential. The central fibrous body provides extensions: (1) the valve rings, to which are inserted the cardiac valves and (2) the membranous interventricular septum,44 that can bulge into the right ventricle to form an aneurysm.
6.2.1 Cardiofibroblasts
Activated fibroblast, a mature cell, synthesizes constituents of the extracellular matrix, such as collagen, elastic, and reticular fibers, as well as glycosaminoglycans and glycoproteins.Fibrocyte, a less active cell type, is involved in matrix maintenance.
Cardiac fibroblasts reside in the cardiac wall, where they align in the direction of myofibers. In the adult human heart, fibroblasts represent about 60% of total cell population, whereas myocytes occupy about 75% of the myocardium volume [568]. They not only synthesize extracellular matrix constituents, but also respond to mechanical and electrical stimuli, and secrete hormones and growth factors involved in cardiac homeostasis.
6.2.1.1 Heterocellular Connections
Heterocellular gap junctions may couple cardiomyocytes and nodal myocytes (especially sinoatrial nodal myocytes) to fibroblasts [568]. Connexin-43 and -45 are produced by both cardiac myocytes and fibroblasts.
In addition, thin, large conductance membrane nanotubes (caliber 50–200 nm), which are ephemeral connections, enable long-distance intercellular interactions between cardiac myocytes and fibroblasts [569]. Membrane nanotubes contain actin filaments and microtubules that can be used for selective molecular transfer. They indeed allow mitochondria exchange and calcium signal propagation between the 2 cell types.
6.2.1.2 Myofibroblast–Myocyte Coupling
Cardiofibroblasts contribute to the regulation of the myocardial function as well as myocardial remodeling in maladaptive hypertrophy of cardiomyocytes during hypertension and after myocardial infarction, among other heart diseases. Myocardial remodeling results from fibroblast proliferation and increased collagen synthesis.
In response to injury, fibroblasts proliferate and differentiate intomyofibroblasts. The latter secrete collagen and synthesize smooth muscle α-actin, stretch-sensitive ion channels, and connexins.
Myofibroblasts can interrupt normal between-myocyte coupling; cardiac fibrosis promotes arrhythmias. Moreover, myofibroblasts can form gap junctions with myocytes, at least in cocultures. The lower negative resting potential of fibroblast plasma membrane can then contribute to depolarization of myocytes. Myofibroblast–myocyte gap junction current comprise an early, transient outward (i K,to)-like component and a late, sustained component. Coupling of myocytes to myofibroblasts promotes early afterdepolarizations due to the former, especially when myocyte repolarization reserve iss reduced by oxidative or ionic stress [570].
6.2.1.3 Effects of Natriuretic Peptides
Numerous growth factors and vasoactive peptides, such astransforming growth factor-β,angiotensin-2, andendothelin-1, participate in maladaptive cardiomyocyte hypertrophy, fibroblast proliferation, and augmented interstitial deposition. Conversely, antagonists of myocyte hypertrophy and fibrosis exist, such as type-A, -B, and -C natriuretic peptides.
Natriuretic peptides, such as ANP and BNP, are released from cardiomyocytes. They then act as auto-, juxta-, and paracrine factors to reduce cardiomyocyte maladaptive hypertrophy and exert antifibrotic and antiproliferative effects on cardiofibroblasts.
Brain natriuretic peptide limits the production of collagen-1 and fibronectin, myofibroblast conversion estimated by α-smooth muscle actin, fibroblast proliferation assessed by PDGFa and IGF1, and inflammation evaluated by COx2 and IL6 levels. C-type natriuretic peptide released from endothelial cells and cardiofibroblasts also has antifibrotic effects.
Cardiofibroblasts synthesizeguanylate cyclase receptors NP1 and NP2 (NPRa and NPRb, or GC2a and GC2b) as well as the natriuretic peptide clearance receptor NP3 (NPRc; Vol. 3 –Chap. 6. Receptors).
All 3 natriuretic peptides trigger the production of cGMP, an inhibitor of cardiomyocyte hypertrophy. Receptor NP3 is involved in the regulation of fibroblast proliferation.45 On the other hand, ANP impedes the activity of ERK and P38MAPK insmooth myocytes in response to angiotensin-2 [571].
Myocardial and coronary development is coupled via bone morphogenetic proteins as well as fibroblast (FGF) and vascular endothelial (VEGFa) growth factors [493].The transcription factor GATA4 regulates cardiac gene expression, thereby modulating cardiomyocyte differentiation and adaptation in adult hearts. In cardiomyocytes,ERK1, ERK2, andP38MAPK kinases phosphorylate (activate) GATA4 transcription factor that promotes myocyte survival and hypertrophy. Factor GATA4 regulates the transcription of numerous peptides synthesized in cardiomyocytes, such as ANP, BNP, α- (MyH6) and β-myosin (MyH7) heavy chains, and endothelin-1 that causes cardiomyocyte hypertrophy [572]. Cardiac lineage-promoting GATA4 factor produced by cardiomyocytes stimulates angiogenic factor VEGFa that targets endothelial cells [573].
Both embryonic and adult cardiofibroblasts produce GATA4 factor. In cocultures of embryonic cardiac fibroblasts and myocytes, the former provoke proliferation of the latter [574].Endothelin-1 serves as an autocrine stimulator of fibroblast proliferation. In cardiofibroblasts, ANP produced in neighboring cardiomyocytes prevents the synthesis of endothelin-1, as it suppresses GATA4-dependent transcriptional activity [571].
6.2.1.4 Effects of Angiotensin-2
Angiotensin-2 is involved in matrix constituent accumulation, especially during cardiac remodeling after long-term exposure of pressure overload. Angiotensin-2 promotes the synthesis ofperiostin, a matrix protein and regulator of cardiac fibrosis [575].46 Angiotensin-2 provokes activation of theRasGRP1–Ras–P38MAPK–CREB pathway.cAMP response element-binding protein responds to different stimuli and mediates the fibrotic response. In addition,ERK1 and ERK2 participate in angiotensin-2-induced periostin expression via the TGFβ1–SMAD2/3 axis.
6.2.2 Telocytes
Telocyte, originally called interstitial Cajal-like cell,47 localizes to the myocardial interstitium, myocardial sleeves of pulmonary veins, as well as subendothelial layer of endocardium [576–578]. This cell differs from other interstitial cell types, such as fibroblasts, fibrocytes, fibroblast-like cells, and mesenchymal cells.
Telocyte is endowed with 2 or 5 long, slender, moniliform (many dilations throughout the length) prolongations, the so-called telopodes (length 0.1–0.4 mm; thickness 0.1–0.5 μm) [578]. These cytoplasmic processes establish close contacts between each other as well as with capillaries, nerve endings, cardiomyocytes, and other connective tissue cells. Telopodes of endocardial telocytes can penetrate the myocardium and extend among the cardiomyocytes and surround them. Telocyte synthesizesstem cell factor receptor [577].
Telocytes may be involved in: (1) intercellular signaling; (2) cardiac repair and remodeling; and (3) stem cell nursing, as it contacts cardiac stem cells in subepicardial cardiogenic niches [579]. A heterogeneous population of round stem and progenitor cells (size 6–10 μm) lodges in mammalian adult heart, more precisely in epicardial stem cell niches. Telocytes make a supportive interstitial network for stem cells and progenitors in the stem cell niche.
6.2.3 Epicardial Adipose Tissue and Adipokines
The epicardial adipose tissue, a source of free fatty acid, generates various substances such as adipokines that include adiponectin, leptin, resistin, apelin, and visfatin. Adipokines exert cardiovascular effects either directly or by central stimulation of the sympathetic nervous system. Epicardial adipose tissue may serve as a cardiac risk marker and be implicated in the development of cardiac pathology [580].
6.2.3.1 Adiponectin
Adiponectin48 (Apn; Vol. 2 – Chaps. 1. Remote Control Cells and 3. Growth Factors and Vol. 3 – Chap. 6. Receptors) produced by adipocytes hascardioprotective effects, via its anti-inflammatory and anti-atherogenic properties (Fig. 6.2). Adiponectin binds to adiponectin receptors AdipoR1 and AdipoR2, which also link adaptors. Adiponectin is an insulin-sensitizing hormone, as it increases sensitivity to insulin in muscle and liver.
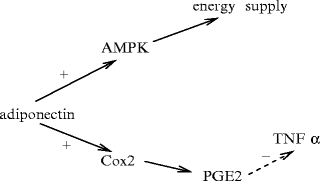
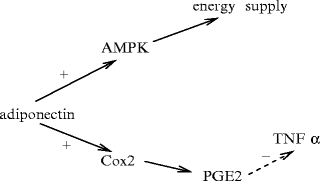
Fig. 6.2
Adiponectin activities. Adiponectin stimulates cyclooxygenase-2 (Cox2) and the synthesis of prostaglandin-E2 (PGE2), and thereby inhibits tumor-necrosis factor TNFα. It also acts via adenosine monophosphate-activated protein kinase (AMPK).
The adiponectin receptors — AdipoR1 and AdipoR2 — are expressed in the heart. Stimulated AdipoRs increasesAMP-activated protein kinase, mediatesperoxisome proliferator-activated receptor-α ligand activities, glucose uptake, and fatty acid oxidation [581]. Low levels of circulating adiponectin, as well as AdipoR1 and -R2, are measured in obesity, which lead to insulin resistance.
In cultured neonatal rat ventriculomyocytes,insulin decreases production of adiponectin receptor AdipoR1, but not that of AdipoR2 receptor via the PI3K–PKB axis andFoxO1 phosphorylation and translocation from the nucleus to the cytosol [582]. Factor FoxO1 binds to the promoter of the ADIPOR1 gene.
Adiponectin acts either via AMPK or viacyclooxygenase COx2, involved in prostanoid synthesis (AMPK-independent pathway; Fig. 6.2). Kinase AMPK, which is also activated by an increased ratio of AMP to ATP, supports fatty acid catabolism and glucose uptake and inhibits glucose synthesis. Enzyme AMPK alsoprotects cardiomyocytes from ischemic injury, particularly hypoxia–reoxygenation-induced apoptosis. Suppression of cardiac AMPK activity indeed leads to increased injury when myocardium perfusion is reduced. Phosphorylation (activation) of AMPK after cardiac ischemia–reperfusion injury is attenuated in hearts of adiponectin-deficient mice [583]. Adiponectin prevents TNFα production by upregulating COx2, and subsequently prostaglandin PGE2. It thus reduces inflammation. Intravenous Apn injection, either shortly before or after the induction of ischemia in mice reduces ischemic injury [583].
6.2.3.2 Leptin
Leptin49 (Vol. 2 – Chaps. 1. Remote Control Cells and 3. Growth Factors and Vol. 3 – Chap. 6. Receptors) is synthesized not only by adipocytes, but also by cardiomyocytes. Leptin production by cultured neonatal rat ventriculomyocytes increases upon stimulation byendothelin-1 andangiotensin-2 [584].
Leptin binds to its receptor (LR, a.k.a. OBR and LEPR) that is expressed as splice variants classified as short (LRa, LRc–LRd, and LRf), secreted (LRe), and long (LRb). Leptin receptors abound on the cardiomyocyte surface. The intracellular domain of LRb primes the JaK2–STAT3 axis. Leptin activates the RhoA–RoCK and PI3K–PKB pathways, as well as ERK1, ERK2, P38MAPK, and PKC [584].
Leptin modulates myocardial metabolism. It operates diversely according to the cell type on fatty acid oxidation and glucose uptake [584].50 Leptin could also limit lipotoxicity that occurs when fatty acid uptake exceeds oxidative capacity. Leptin has negative inotropic effect via nitric oxide. Leptin is a predictor of poor outcome in patients with coronary heart disease or heart failure [584]. Leptin induces cardiac hypertrophy via the RhoA–P38MAPK pathway.
6.2.3.3 Resistin, Apelin, Visfatin, and Other Adipokines
Apelin binds to G-protein-coupled APJ receptor and exerts a positiveinotropic effect in both normal and failing myocardium viaprotein kinase-C andNa + –H + exchangers [584]. Apelin exerts acardioprotection against ischemia–reperfusion injury.
Resistin is produced almost exclusively in white adipose tissue. It circulates as a trimer (monomeric form of resistin) or hexamer (dimeric form). Resistin is associated with insulin resistance. The dimerized form of resistin is more effective in antagonizing insulin-stimulated glucose uptake in adult murine cardiomyocytes [584].
Visfatin associates with the insulin receptor to induce hypoglycemia. Visfatin level is elevated in patients with coronary artery disease [584]. Other adipokines comprise vaspin, omentin, and chemerin, but they do not have identified function in healthy or diseased heart.
6.2.4 Heart Valves
The cardiac valves are sheets of connective tissue that begin at the annulus fibrosus. The valve cusps are passive soft tissues attached to the wall at the insertion line.
The ventriculoarterial — aortic andpulmonary — valves have smooth ventricular and wavy arterial faces. The free edge is indented. The middle part of the free edge is thicker with the Arantius nodule, characterized by a high concentration of collagen fibers.
These geometric features influence both valve motion during the cardiac cycle,51 as well as flow in the aortic sinus when it is open, and thus the transvalvular pressure. Effective boundary conditions or wall laws for laminar pattern can be introduced. Roughness elements are supposed to be periodic with a length scale greater than the flowing cell size [585].
The cardiac valves, covered by anendothelium and containingsmooth myocytes, are reinforced by many internal bundles of collagen and elastic fibers (Table 6.5). Sparsely distributed valvular interstitial cells have extensive contact with proteoglycans of the extracellular matrix, as well as with elastin and collagen fibers.
Table 6.5
Structural elements of heart valves, with the 3 layers: (1) the ventricularis (rich in elastin); (2) the central spongiosa; and (3) the fibrosa (with packed collagen). Forces applied on the valve leaflets (pressure, shear, tension, and bending) are borne by structural rearrangement (fiber reorientation, folding, uncrimping, and compaction). Collagen and elastin fibers cooperate. Alignment of packed collagen bundles transfers forces between the cusps and the wall. Corrugations of the cusp face in front of the wall (fibrosa) help size and shape changes during the cardiac cycle. Microscopic collagen foldings (crimps) enable lengthening from low stresses. The anisotropic behavior is responsible for required differences in radial and circumferential extensibility. Cusp coaptation prevents valve prolapse. The trileaflet semilunar ventriculoarterial valves are inserted and supported by aortic and pulmonary roots. The atrioventricular valves are inserted on an annulus. Chordae tendineae and papillary muscles maintain the atrioventricular valve closed during blood ejection from the ventricles into the corresponding arteries (Source: [587]).
Component | Function |
---|---|
Cells | Interstitial cells synthesize and remodel ECM |
(fibroblasts, smooth myocytes, myofibroblasts) | |
Valvular endothelial cells regulate the behavior | |
of flowing and mural cells | |
Matrix | Elastin fibers extend in closed valve |
and shrink in open valve | |
Collagen fibers maintain coaptation in closed valve | |
Proteoglycans associate with matrix fibers | |
for stress accommodation | |
Blood vessels | Valve cusps are avascular |
Nutrient/waste transport from heart cavity | |
and myocardial vessels | |
Nerves | Regulation of coordinated activity |
of heart components |
Collagen-1 forms a dense content beneath theendothelium. Collagen fibers of the outer layer of the cusp are mainly oriented in the azimuthal direction. Collagen fibers are more randomly oriented in the cusp central part.Elastin fibers are more abundant near the fibrous ring. In the fibrous ring, collagen fibers form massive wavy twisted bundles and elastin fibers have different directions. Also in the commissures, collagen fibers form massive twisted bundles.
Chordae tendinae mingle with valvular connective tissue. The myocardium penetrates into the valve leaflets. The valve components and their estimated elastic moduli are given in Vol. 7 – Chap. 5. Rheology. Whatever the transvalvular pressure at which the porcine aortic valves are fixed ( < 12 kPa), the fibers mainly run along the circumferential direction [586]. Moreover, strong collagen fiber bundles that travel through the valve cusps and attach to the arterial walls behave like suspension cables for force transmission.
The cusp is a multilayer structure. The 3 layers are the fibrosa, the spongiosa (which is absent in the coaptation region), and the ventricularis (Fig. 6.3). The fibrosa, located toward the arterial wall, contains a large number of circumferential52 wide collagen bundles. These circumferential bundles are arranged in a corrugated manner for radial expansion. A matrix with elastin surrounds the collagen bundles. The ventricularis contains collagen and elastin fibers, which are less organized than in the fibrosa. However, elastin sheets provide recoil, which retains the folded shape of the fibrosa. The loosely organized spongiosa, between the fibrosa and ventricularis, contains glycosaminoglycans, collagen, and elastin mostly in the radial direction. The spongiosa could act as load buffer for the fibrosa and the ventricularis.
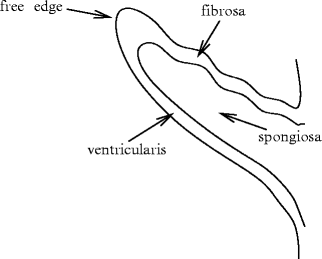
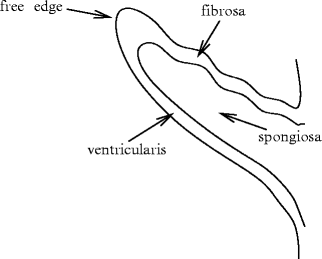
Fig. 6.3
Ventriculoarterial valve layers, according to the internal collagen network of the valve leaflets: fibrosa, spongiosa and ventricularis from the wavy arterial face to the smooth ventricular face. Collagen fibers provide strength, elastin fibers elasticity and proteoglycans swelling. Collagen architecture is mediated by mechanical loading.
The heart structure provides the 3 properties of contractility, automatism, and conduction due to 2 types of cardiac muscular cells: myocardial and nodal myocytes.
6.2.4.1 Mathematical Histology
Structure–function features of the heart have been mathematically investigated. Two fiber networks have been particularly studied: (1) the network of collagen fibers of the aortic valve cusp, and (2) the myofibers of the left ventricle wall, using a simple model of mechanically loaded fibers. The structure of the aortic leaflet has been derived from its function, which is assumed to consist of supporting a uniform pressure load undergone by a single family of fibers under tension [588]. The equation of equilibrium for the fiber structure is solved to determine its architecture. The computed fiber architecture resembles the real one. Assuming a constant myofiber cross-sectional area, symmetry with respect to the ventricle axis, small wall thickness with respect to the other dimensions, and a stress tensor resulting from hydrostatic pressure and myofiber stress, the bundles of myofibers have been shown to be located on approximate geodesics on a nested set of toroidal surfaces centered on a degenerate torus in the equatorial plane of the cylindrical part of the left ventricle [589].
6.2.5 Conduction Paths of the Depolarization Wave
The sinoatrial node act as the primary cardiac pacemaker. Together with nodal cells in the atrioventricular node (secondary cardiac pacemaker) and those of the ventricular nodal circuit, they constitute the cardiac conduction network of electrochemical waves (or action potentials).
6.2.5.1 Nodal Cells
Nodal cells are small (80–100 ×10–20 μm), specialized cardiomyocytes with few myofibrils that create or quickly spread depolarization waves in the myocardium. The conducting cells are wider than actual cardiomyocytes. They are not branched.
In the absence of neural and hormonal control, nodal cells naturally create action potentials, i.e., electrochemical signals at a given frequency that varies along the nodal tissue. When an upstream element becomes dysfunctional, a group of cells in a downstream part of the nodal tissue takes the relay, but at a lower frequency.
Tight junctions (Vol. 1 – Chap. 7. Plasma Membrane) facilitate action-potential propagation. Gap junctions also transmit electrochemical signals in the excitable myocardium, in which response speed and tissue activity synchronization are crucial for ion transfer.
Nodal myocytes are sparse with dispersed gap junctions that have high-conductanceconnexin-40 and low-conductance connexin-45.53Nodal Purkinje cells have abundant gap junctions with connexin-40 and -45 [333].
6.2.5.2 Architecture of the Nodal Tissue
Nodal cell automaticity relies on spontaneous, rhythmic, local depolarization associated with ionic fluxes. The sinoatrial node (SAN) that has the highest emission frequency of action potentials (Table 6.6) constitutesthe “natural pacemaker”.
Table 6.6
Auto-excitation frequency (Hz) in nodal tissue (AVN: atrioventricular node; SAN: sinoatrial node). SAN frequency is decreased by the vagal influence (cranial nerve X).
SAN | 2.0– ![]() |
AVN | 0.5–1 |
His bundle | 0.3–0.6 |
Purkinje fibers | 0.2–0.5 |
The nodal tissue permits electrochemical conduction through the heart from the sinoatrial node, both atria, to the atrioventricular node. Electrochemical signals then propagate across the atrioventricular septum and ventricles using the His bundle and its 2 branches — left and right bundle branches — that ramify into Purkinje fibers organized in many fascicles. The latter transmits the depolarization wave to the inner layers of cardiomyocytes. Afterward, the electrochemical wave spreads through the surface and the width of the ventricular myocardium in a given time.
An automatism hierarchy exists between different regions of nodal tissue, according to the emission frequency of the action potential (Table 6.6). The propagation speed of the electrochemical signal also varies with the heart region (Table 6.7).
Table 6.7
Estimated conduction speeds (m/s) in nodal tissue (AVN: atrioventricular node; SAN: sinoatrial node).
SAN | 0.05 |
Atrium | 0.3–1 |
AVN | 0.02–0.10 |
His bundle | 1–2 |
Purkinje network | 3–5 |
Ventricle (axial) | 0.6–1 |
Ventricle (transverse) | 0.2–0.5 |
6.2.5.3 Sinoatrial Node
The electrochemical signal starts with automatic self-excitation of cells of the sinoatrial node (SAN; length ∼ 8 mm, width ∼ 2 mm). This node is located at the top of the right atrium, more precisely at the junction of the superior vena cava and right atria, between the orifice of the superior vena cava and the auricle. In the majority of humans, the sinoatrial node is irrigated by the right coronary artery.
The sinoatrial node is composed of different cell types, stellate and elongated spindle cells. Stellate myocytes, the primary pacemaker cells, exhibit a faster functioning rate and longer action potential duration.
In adult mouse hearts, the density ofconnexin-30 is low in the sinoatrial node, where it contributes to the regulation of the cardiac frequency, in addition to hyperpolarization-activated cyclic nucleotide-gated potassium channel HCN4 and other ion carriers [591].
The sinoatrial node is endowed by conduction barriers to be functionally insulated from the surrounding atrial myocardium as well as conduction paths to transmit action potentials. Anatomical barriers, i.e., connective tissue layers, adipose tissue, and coronary arteries, as well as functional barriers, which results from gap junction connexin paucity, protect from hyperpolarizing influence of the surrounding atrial cells [592]. Specialized paths — sinoatrial conduction pathways — represent preferential lines of conduction of action potentials. They transmit electrochemical impulses from the sinoatrial node to the atrial wall. They consist of elongated and branching fibers of transitional cells.
In dogs, the sinoatrial node, which is a more realistic animal model for the human SAN than that of small mammals, is isolated from the surrounding atrium by 3 branches of the coronary arterial bed and fibrosis [592].
The sinoatrial node is a heterogeneous structure with many pacemaker compartments and conduction pathways, electrophysiological properties of which vary. Major pacemaker compartments comprise head, center, and tail of the sinoatrial node [592]. A pacemaker hierarchy within the SAN exists with the fastest pacemakers at the head and the slowest at the tail. Electrochemical coupling enables the synchronization of pacemaker clusters inside the sinoatrial node. Excitation originates in the quickest pacemaker compartment and slowly propagates through the SAN tissue. It then runs via different conduction paths and excites atria (beginning of the P wave on the electrocardiogram).
Both canine and human sinoatrial nodes have at least 4 preferential conduction paths, such as the superior and inferior conduction paths, that deliver depolarization waves simultaneously to different regions of the atrial myocardium. According to the preferential conduction paths, action potential spreads from the right atria to the left atria either via the Bachmann’s bundle and the coronary sinus myofibers or via anterosuperior and posteroinferior intra-atrial septum connections [592].
In addition to hyperpolarization-activated inward “funny” current (i f) through hyperpolarization-activated cyclic nucleotide-gated channels, various other inward currents can contribute to cardiac automaticity, such as i NaCaX current throughNa + –Ca2 + exchanger (calcium clock mechanism). Blockers of i f slow, but do not stop automaticity, whereas ryanodine that depletes intracellular Ca2 + stores can cause a cessation of automaticity. The Ca2 + influx regulates Na + –Ca2 + exchanger. Spontaneous, cyclical, local Ca2 + release from the sarcoplasmic reticulum can indeed participate in the generation of both basal and adrenergically stimulated depolarization rate, in addition to i f current [593]. In fact, Ca2 + dependence of SAN automaticity, i.e., the calcium clock, is directly and indirectly linked to the plasmalemmal ion flux mechanism of SAN automaticity. The sinoatrial node produces a Ca2 + -stimulatedadenylate cyclase (AC1 or AC8) rather than the typical cardiac isoform (AC5 or AC6).
6.2.5.4 Atrial Paths
Since sinoatrial fibers fuse with the surrounding atrial cardiomyocytes, the action potential spreads through the atria at a rate at least of ∼ 0. 3 m/s and produces atrial contraction.
However, several nodal bundles conduct action potentials with a greater speed of about 1 m/s. Three preferred conduction paths have been observed in the right atrium wall: the anterior Thorel, the mid Bachman, and the posterior Wenckebach bundles.
6.2.5.5 Atrioventricular Node
The action potential reaches theatrioventricular node (AVN; ∼ 5 mm long) that localizes to the right atrium near the lower part of the interatrial septum. The atrioventricular node is adjacent to the coronary sinus.
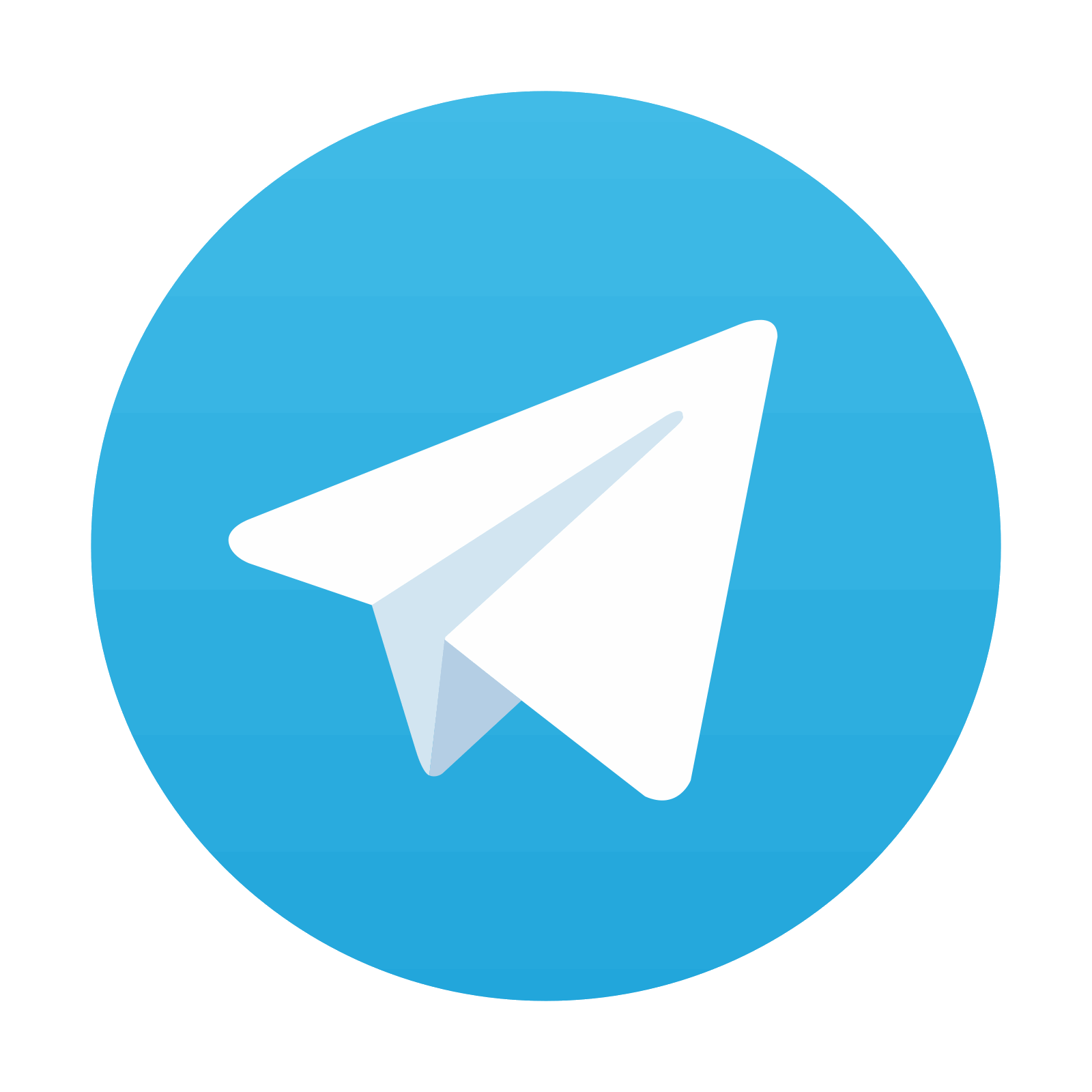
Stay updated, free articles. Join our Telegram channel
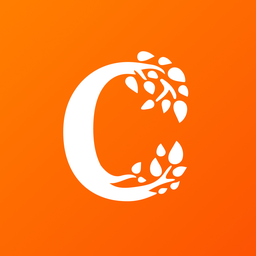
Full access? Get Clinical Tree
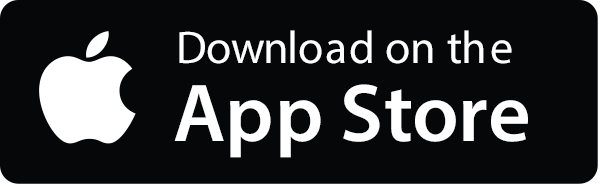
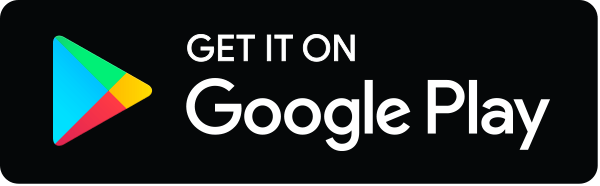
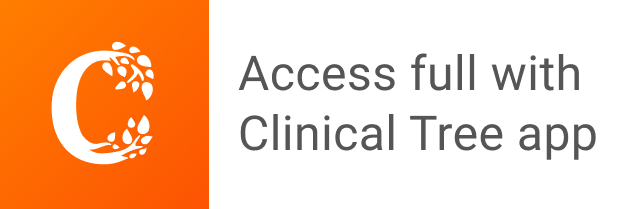