Recent progress in the area of cardiovascular genetics has led to a better understanding of the pathogenesis of inherited arrhythmia syndromes, also referred to as channelopathies. Mutations in genes encoding for specific ion channels have been shown to cause specific forms of heritable arrhythmia disorders occurring in the structurally normal heart. Figure 51.2 and Table 51.1 summarize the common genes and proteins associated with common inherited arrhythmia syndromes. Current guidelines recommend genetic counseling or mutation-specific genetic screening of first-degree relatives of patients with inherited arrhythmia syndromes to identify affected family members, caused by increased risk of adverse cardiac events in genotype-positive patients.4
![]() FIGURE 51.1 Cardiac action potential and respective ion currents during various phases. IK1, inward rectifier K current; INa, Na current; Ito, transient outward K current; IKur, ultrarapid component of the delayed rectifier K current; IKr, rapid component of the delayed rectifier K current; IKs, slow component of the delayed rectifier K current; NCX, Na-Ca exchanger; If, hyperpolarization-activated nonselective pacemaker “funny” current; IKACh, Acetylcholine-activated K current. (Reprinted by permission from Nature: Nattel S, Carlsson L. Innovative approaches to anti-arrhythmic drug therapy. Nat Rev Drug Discov. 2006;5(12):1034-1049. Copyright © 2006 Springer Nature.) |
![]() FIGURE 51.2 Genes and proteins involved in the pathogenesis of hereditary cardiac arrhythmias. The genes that encode ion channels and proteins in the sarcoplasmic reticulum are color-coded according to the inherited arrhythmia disorder in which they are implicated. BrS, Brugada syndrome; CPVT, catecholaminergic polymorphic ventricular tachycardia; LQTS, long QT syndrome; SQTS, short QT syndrome. Reprinted with permission from Schwartz PJ, Ackerman MJ, Antzelevitch C, et al. Inherited cardiac arrhythmias. Nat Rev Dis Primers. 2020;6:58. |
TABLE 51.1 Genes Implicated in Inherited Cardiac Arrhythmia Syndromes | |||||||||||||||||||||||||||||||||||||||||||||||||||||||||||||||||||||||||||||||||||||||||||
---|---|---|---|---|---|---|---|---|---|---|---|---|---|---|---|---|---|---|---|---|---|---|---|---|---|---|---|---|---|---|---|---|---|---|---|---|---|---|---|---|---|---|---|---|---|---|---|---|---|---|---|---|---|---|---|---|---|---|---|---|---|---|---|---|---|---|---|---|---|---|---|---|---|---|---|---|---|---|---|---|---|---|---|---|---|---|---|---|---|---|---|
|
only modestly prolonged QTc intervals, with such cases being detected during mandatory screening prior to participation in sports. Secondary causes of QT prolongation (such as medications, disease states, or electrolyte disturbances) must be excluded. Exercise testing should be performed to assess for exercise-induced arrhythmias (although rare in LQTS), changes in T wave morphology, and presence of a maladaptive QT response during the recovery phase. Ambulatory rhythm monitoring can provide supportive information, including intermittent QT prolongation and underlying dynamic T wave changes, especially at night. The LQTS diagnostic score, also known as the Schwartz Score,9 first developed in 1985 and most recently updated in 2011, should be calculated (Table 51.2). A high probability Schwartz score (≥3.5 points) carries ˜80% likelihood of a positive LQTS genetic test. Genetic testing should not be pursued in patients with a low Schwartz score (<1 point). The likelihood of LQTS is ˜5% to 20% for an intermediate probability Schwartz score, and negative genetic testing in this situation would not warrant a diagnosis of LQTS. Genetic testing not only aids in the diagnosis of LQTS in the presenting patient but also helps identify asymptomatic but at-risk family members who may not have been otherwise diagnosed and received life-saving therapy.1
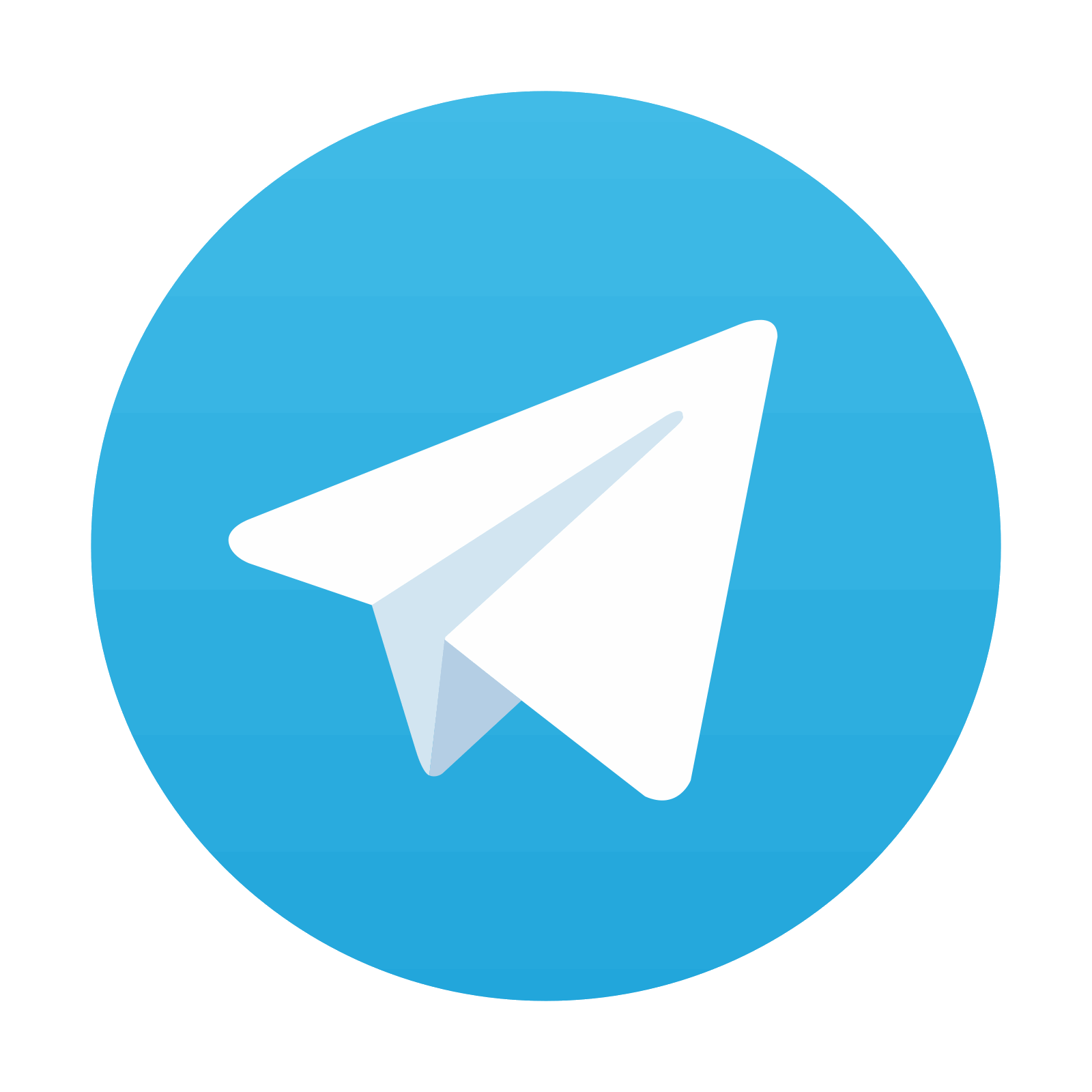
Stay updated, free articles. Join our Telegram channel
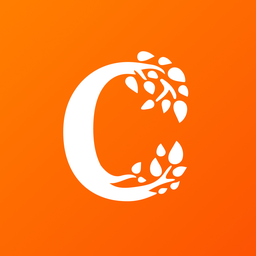
Full access? Get Clinical Tree
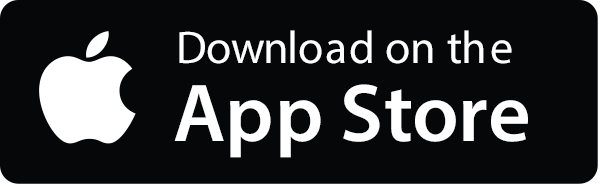
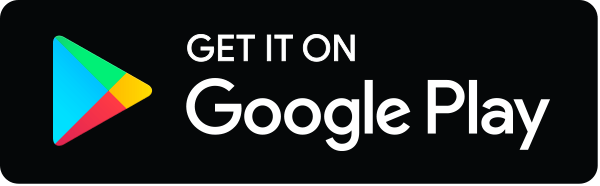
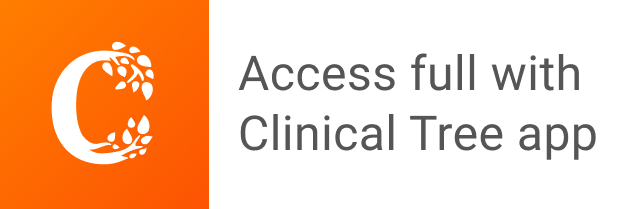