, Rohit Arora3, 4, Nicholas L. DePace5 and Aaron I. Vinik6
(1)
Autonomic Laboratory Department of Cardiology, Drexel University College of Medicine, Philadelphia, PA, USA
(2)
ANSAR Medical Technologies, Inc., Philadelphia, PA, USA
(3)
Department of Medicine, Captain James A. Lovell Federal Health Care Center, North Chicago, IL, USA
(4)
Department of Cardiology, The Chicago Medical School, North Chicago, IL, USA
(5)
Department of Cardiology, Hahnemann Hospital Drexel University College of Medicine, Philadelphia, PA, USA
(6)
Department of Medicine, Eastern Virginia Medical School Strelitz Diabetes Research Center, Norfolk, VA, USA
Parasympathetic Excess: Diagnosis and Therapy
A common assumption with Autonomic (P and S) Assessment is one branch opposes the other. With independent measures of P&S activity (P&S monitoring), this assumption has been challenged and is no longer required. Clinical observations of unprovoked PE during sympathetic stimulation have been associated with abnormal responses. Serial autonomic profiling of 1,340 patients was performed using P&S monitoring over a 3-year period. PE patients present with normal HR and BP and no other apparent causes. However, they reported symptoms of sleep disturbances, poor peripheral circulation, general malaise, depression, frequent headache or migraines, gastrointestinal upset, or dizziness upon standing and often demonstrate difficult to control BP, blood glucose, or hormone level (e.g., thyroid or estrogen).
Elsewhere in the literature, the symptoms associated with PE (as measured during stress or exercise or a sympathetic challenge) are similar to what Levine’s lab calls “cardiovascular deconditioning” [1–4]. In patients with symptoms, PE is associated with difficult-to-control BP, difficult-to-control blood glucose, or difficult-to-control hormone levels. Treatment protocols depend on patient history. For cardiovascular disease (CVD) patients, consider carvedilol, history dependent. For non-CVD patients, consider low-dose anticholinergics. In some cases where end-organ effects are not yet presented, patients may be weaned of therapy once PE is resolved.
This manuscript was first published as an article in US Neurology, 2010, entitled Autonomic Nervous System Monitoring of Patients with Excess Parasympathetic Responses to Sympathetic Challenges – Clinical Observations [5]. Excerpts of this manuscript are presented here.
The assumption regarding the oppositional activity of the two autonomic branches is required by the HBI-alone measures. The reason being that these measures provide only information regarding the health or response of the whole ANS. Even if two of these tests, one for S and one for P, are performed back to back, the assumptions are still forced, due to only one branch being stimulated and assumed measured at a time. Due to the dynamic nature of the ANS and the continuous interaction between P&S, simultaneous, independent P&S measures are required to document PE and fully understand autonomic activity in individual patients.
Clinical observations of unprovoked parasympathetic activity during a sympathetic challenge, hereinafter referred to as PE, and abnormal sympathetic activity with P&S measures have been associated with abnormal clinical and pathophysiological symptoms. Clinically, PE is associated with chronic or severe acute conditions. It is known that chronic diseases such as diabetes [6–10], thyroid diseases [11], kidney diseases [12], cardiovascular diseases [7, 9], demyelinating and inflammatory neurological diseases [13], some dementia [14], depression, and altered psychological states [14] may cause autonomic imbalance, including parasympathetic dysfunction. Severe acute conditions may precipitate PE, including trauma or pain (including CRPS), injury, infection, surgery (including CABG), cancer, and MI. Preliminary evidence suggests that multiple pregnancies may also leave the mother with PE. Severe or chronic chemical, cold, and allergen (e.g., mold) exposure may affect PE. It is also known that lifestyle may affect autonomic balance such as stress, excess caffeine or nicotine, or other chemical [15, 16] exposures. Genetically mediated PE may be evidenced by colic in infants.
PE may clarify patient reports of multiple symptoms (e.g., hypertension with depression) and help to provide a more integrated approach to therapy. Given the fact that disease or disorder causes autonomic imbalance (e.g., chronic disease like pain or diabetes causing SE leading to early hypertension) or is caused by autonomic imbalances (e.g., dizziness from sympathetic abnormalities while standing), it seems reasonable to hypothesize that autonomic imbalances such as PE are separate and distinct dysfunctions. Often, a single agent addresses both the primary and autonomic disorders. If not, PE may be treated concurrently with the primary disorder. Either way, once PE is corrected, often the patient is more stable and the primary disease(s) may be treated more aggressively.
P&S monitoring documents failures in the reactive push–pull dynamics within the ANS. P&S monitoring is critical in our understanding of the true nature of autonomic dysfunction and its clinical implications. Simultaneous, independent documentation of P&S activity has helped to provide more insight into many commonly observed clinical conditions. The PE seems to be the primary autonomic disorder and the (reactionary) sympathetic abnormalities seem to be secondary.
Background
PE presents in response to sympathetic challenge (e.g., Valsalva or stand). For example, any sort of parasympathetic increase in response to head-up PC is considered abnormal. Normally, parasympathetic activity decreases first, potentiating the sympathetic increase that follows to perpetuate the expected vasoconstriction required to defeat orthostasis. The parasympathetic decrease also (normally) minimizes the sympathetic increase required. Similarly, stress responses, such as short Valsalva maneuvers (less than 15 s), are expected to cause a decrease in average parasympathetic activity. An (abnormal) increase in parasympathetic activity to either of these sympathetic challenges seems to force a higher sympathetic response than typical for that patient and condition. These (relative) SEs often lead to increases in BP or HR. The PEs may also lead to unexplained arrhythmia. With this symptomatology, it is no wonder that PE patients are treated as if the patient demonstrated a primary SE. Yet patient responses under these autonomic conditions are unexpected or paradoxic. By definition, PE is a dynamic autonomic imbalance, as opposed to a resting imbalance. PE response to sympathetic challenge will be referred to as a “dynamic PE,” or simply PE.
Understanding the physiology of some of these clinical assessment challenges helps to understand PE and its clinical implications. The short Valsalva maneuver is normally a significant net sympathetic challenge and should have little or no net parasympathetic effect. Valsalva simulates normal stresses that occur daily, such as exercise, bowel movements, domestic and office stresses, and other stresses, such as “road rage.” Valsalva challenge helps to clinically differentiate hypertensives that are truly normalized from those who are only normotensive at rest and still at risk of stroke or MI due to SE with exertion. PE as measured during the Valsalva challenge, regardless of whether SE is primary or secondary, is abnormal. The predominant sympathetic response to Valsalva is a beta-adrenergic response.
The head-up PC challenge is actually a challenge to both ANS branches, a test of the coordination between the two. However, since the parasympathetics are expected to decrease and the sympathetics are expected to increase, the commonly perceived net effect is that PC is a sympathetic challenge. The predominant sympathetic response to PC is an alpha-adrenergic response. In this way, it is possible to detect both a SE (e.g., in response to Valsalva) and a sympathetic insufficiency (e.g., in response to PC) from the same autonomic assessment.
Normal P&S Responses to a Valsalva Maneuver
Figure 14.1 depicts the instantaneous HR (red) and respiratory activity (RA, blue) responses of a normal subject during a Valsalva maneuver. With the initiation of a Valsalva maneuver (Fig. 14.1, #1 HR), there is a sudden increase in intrathoracic pressure. This mechanical pressure increase is falsely sensed by the baroreceptors at the level of the heart as a sudden increase in BP (due to an increase in cardiac output). In actuality, the Valsalva maneuver lowers cardiac output by shunting blood away from the heart. The Valsalva maneuver is initiated by inhalation (Fig. 14.1, #1 RA), causing parasympathetic (vagal) inhibition, which is immediately followed by an increase in HR (Fig. 14.1, #1 HR) from the cardiovagal inhibition as stretch receptors in the lungs are unloaded. The sudden increase in intrathoracic pressure stimulates the thoracic baroreceptors, causing a momentary parasympathetic response, and HR drops (Fig. 14.1, #2 HR). As blood is shunted from the thorax, two reflexes, the baroreceptor reflex and the venoarteriolar axon reflex, stimulate sympathetic activity.


Fig. 14.1
The respiratory and HR responses to the Valsalva maneuver. The vertical broken lines indicate the phases of P&S activity during the Valsalva maneuver
Due to decreased venous return to the heart, there is a pressure drop at the aorta caused by a decrease in cardiac output (and the baroreceptors are unloaded). In addition, there is no opposing peripheral vasoconstriction (no preexisting sympathetic activity upon initiation of the Valsalva maneuver). So, as blood is shunted freely into the peripheral vessels, the venoarteriolar axon reflex is initiated as the transmural pressure within the blood vessels begins to exceed 25 mmHg. As a result, sympathetic activity is stimulated and there is a gradual increase in HR (Fig. 14.1, #3 HR).
Upon release of Valsalva during exhalation (Fig. 14.1, #4 RA), there is an overshoot of BP resulting from the sudden rush of blood back to the heart. This overshoot is compounded by the residual sympathetic activity (causing peripheral vasoconstriction) exaggerating the blood return into the thorax and opposing venous return into the extremities. The baroreceptors in the thorax sense this sudden stretch, causing a parasympathetic surge to inhibit the sympathetic activity and start to return HR to normal. Immediately following the release of the Valsalva maneuver, the HR continues to increase for a short period of time for two reasons. First, sympathetic activity, which is slower to react, continues to rise, so there is a short period of residual sympathetic activity. Second, with release of the Valsalva maneuver, the natural response is to inhale deeply (Fig. 14.1, #5 RA), causing parasympathetic (vagal) inhibition, causing the HR to continue to rise (Fig. 14.1, #5 HR). After the release of the Valsalva, HR and RA finish returning to normal (Fig. 14.1, #7) and normal sinus rhythm, synchronized with the respirations, continues until the end of the recording (Fig. 14.1, #8) [17].
Thus, in normal subjects, short Valsalva maneuvers are expected to create a significant rise in sympathetic activity with little if any net increase in parasympathetic activity, especially when averaged over the duration of the course of the Valsalva challenge during P&S monitoring (Fig. 14.2, graph section D). From the table in the insert, the subject’s sympathetic activity (LFa) increases 6,088 % from Baseline (A) to Valsalva (D), whereas the parasympathetic activity (RFa) increases by only 26.20 %. The trend plot in the insert shows the instantaneous values. Notice the large surge in sympathetic activity (red trace) during the Valsalva challenge (D: a series of five short Valsalva maneuvers) with only a relatively small increase in parasympathetic activity (blue trace). The desire to minimize parasympathetic activity is the reason technicians are trained to keep the Valsalva breaths as short as possible to prevent false positives for PE.


Fig. 14.2
The average (see the table) and instantaneous (see trend plot) normal responses to the six autonomic assessment phases
Normal ANS Responses to a Rapid Postural Change
PC is not as stressful as Valsalva; therefore, average or instantaneous sympathetic responses to stand (see Fig. 14.2, graph section F) should not be as great as those for Valsalva (graph section D). For PC, an average parasympathetic decrease from rest to PC is expected (see Fig. 14.2, Table: “RFa” changes from 1.87 to 0.74 bpm2, row “A” to “F,” respectively). If, in fact, there is a PC SE or PC PE, the physician is given insight into a possible cause of dizziness (possible syncope or orthostasis). Increases in parasympathetic activity during PC may defeat or mask the sympathetic surge, decreasing the expected vasoconstriction or possibly causing vasodilatation, leading to an inappropriate gravitational displacement of blood to the legs. Thus, PC poses a major challenge to circulatory homeostasis, mandating that the autonomic control center maintain arterial pressure and cerebral perfusion irrespective of the effect of gravity [17].
Figure 14.3 depicts the instantaneous HR (red) and RA (blue) responses of a normal subject during PC. Upon assuming a head-up posture (Fig. 14.3 #1 RA) and maintaining normal breathing throughout, there is a large shift in blood from the thorax to the abdomen and lower extremities, with a corresponding decrease in cardiac output. These changes provide a strong sympathetic stimulus. The fluid shift upon standing is immediate, and there is initially an abrupt increase in HR (in the first 3 s, Fig. 14.3 #1 to #2, HR) followed by a more gradual increase (occurring in the next 3–12 s, Fig. 14.3 #2 to #3, HR).


Fig. 14.3
The respiratory and HR responses to the postural change (stand) maneuver. The vertical broken lines indicate the phases of P&S activity during the stand maneuver
Since the sympathetics take three to five heartbeats to respond [17], this means that the initial HR increase is not due to sympathetic activity. It is due to a combination of parasympathetic inhibition and the exercise reflex. The exercise reflex is initiated as the leg muscles are engaged with PC. The leg muscles manually clamp down on the peripheral blood vessels, helping to maintain blood in the abdomen and supporting the heart. This is supported by parasympathetic inhibition, potentiating the expected sympathetic surge by causing a relative sympathetic dominance. Then, as the sympathetics respond and initiate peripheral vasoconstriction, the gravitational effect on the blood while standing is opposed. The absolute amplitude of the sympathetic response is minimized by the parasympathetic inhibition. Following the exercise reflex, an additional sympathetic stimulus, the venoarteriolar axon reflex, is realized. The venoarteriolar reflex is thought to account for 40 % of the peripheral vascular resistance during stand.
Sympathetic activation is seen as a gradual increase in HR (Fig. 14.3 #2 to #3, HR). Sympathetic activation maintains cardiac filling pressure by constriction of splanchnic (visceral) capacitance vessels. As peripheral vascular resistance increases, core sympathetic discharge stimulates core parasympathetic neurons. Finally, a saturation point is reached within the baroreceptors, and the parasympathetics normalize HR to a new baseline level (Fig. 14.3 #4 in insert, HR). Hemodynamic PC responses include a 10–30 % increase in mean HR and a 0–10 % increase in mean arterial pressure (MAP). This is due to the fact that the baroreceptors located in the carotid artery (transporting blood to the brain) now sense a lower capillary pressure than during sitting. This pressure differential provides the stimulus, which maintains increased sympathetic activity while maintaining upright posture. (The feedback is blunted with PE.) The net normal result is an expected decrease in parasympathetic activity followed by an increase in sympathetic activity with an increase in HR, BP, and a reflexive increase in depth of respiration in nonobese patients [17].
Methods
Serial autonomic profiling of 1,340 patients (746 females, 55.7 %) was performed using the P&S monitoring (see Chap. 5) over a 3-year period at six primary care, ambulatory clinics located in six different states along the eastern seaboard of the United States. Patients were followed as a matter of routine, based on their primary diagnosis. The results were analyzed and statistics were computed using SPSS v14.0.
Treatment Protocols
Treatment protocols were developed based on the following observations. The parasympathetics establish and regulate metabolic thresholds to which the sympathetics react or respond. The upper medullary brain stem nuclei, the nucleus solitarius, the dorsal nuclei of the vagus nerve, and surrounding small nuclei that give rise to the vagus nerve link the mind and body and are the core of the ANS. The lack of proper venous tone plays an important role in clinical manifestations of autonomic dysfunction and supports a positive feedback loop for persistent autonomic imbalance leading to more symptoms. Abnormal sympathetic responses may be a secondary phenomenon and do not always require treatment. Various central nervous system drugs and vasoactive drugs (e.g., reticular activating system drugs and vasodilators) may interfere with ANS therapy and adversely affect the ANS (e.g., peripheral β-blockers, ACE inhibitors, and systemic vasodilators).
Figure 14.4 depicts the generalized closed-loop connections of ANS regulation, highlighting its central and peripheral influences. Three treatment protocols for PE were developed. For patients with cardiovascular disease (CVD, including hypertension) with PE, low-dose carvedilol (or dose equivalent if a beta-blocker is already prescribed) should be considered, history dependent. The beta-adrenergic antagonist within carvedilol addresses the CVD and the alpha-adrenergic antagonist, which is known to cross the blood–brain barrier, seems to indirectly address the PE [18–22]. For PE patients without CVD, low-dose anticholinergic therapy (e.g., amitriptyline or nortriptyline, starting with 10 or 12.5 mg QD, dinner) or duloxetine (e.g., 20 mg) often reduces systemic parasympathetic activity. In many cases, PE masks SW, and SW has been found to lead to high BP [23] and other symptoms. For SW, proper daily hydration is recommended, along with reducing any diuretics. In patients with well-managed BP (resting BP <160/90) and no supine hypertension, consider low-dose midodrine (e.g., starting with 2.5 mg QD, dinner) [23]. Often, carvedilol with midodrine are prescribed in patients with CVD and SW. The carvedilol first and the midodrine (as needed) if and when dizziness is reported. It helps to encourage the patient that the dizziness is a good sign. It means their ANS is responding to therapy. Consider short-term therapy based on correction of PE or SW. The term is based on the duration of the disorder and may be between 9 and 18 months. The ability to wean is based on the plasticity of the patient’s ANS.


Fig. 14.4
The central feedback point between the PSNS and SNS in the upper medulla. Also depicted are the sites of action of autonomically active medications
Alternative therapies include exercises that do not incur any tissue damage, such as swimming, long gentle walks, rowing, simulated cross-country skiing, or elliptical exercise machines. Exercises to be avoided include running or jogging, weight-lifting, and contact sports.
Results
Patients with PE present with normal HR and BP and no other apparent causes from the standard physical. In fact, 2.2 % of the cohort was initially diagnosed as healthy. The average resting HR for the cohort is 72.7 bpm (±13.1 bpm). The average BP for the cohort is 132.3/71.9 mmHg. According to current AHA guidelines [24], the patients ranging in age from 60 to 90 had mild hypertension (average BP = 140.7/66.6 mmHg).
PE appears to destabilize patients’ responses to disease and therapy and is associated with difficult-to-control BP, blood glucose, and hormone levels (e.g., thyroid, estrogen, and growth hormones). There are 58.5 % of the population diagnosed with hypertension, including 21.9 % reported with difficult-to-control BP or labile hypertension. Those diagnosed with diabetes mellitus, either type I or type II, comprised 51.8 % of the cohort, with 19.8 % reporting difficult-to-control blood sugars. Those diagnosed with hypothyroid disorders comprised 15.3 % of the cohort, with 8.1 % reporting high levels of hormone replacement therapy and complaints of significant, continuing secondary symptoms. Of the female population within this cohort, 19.1 % reported menstrual or menopausal abnormalities.
PE is associated with symptoms of (1) sleep disturbances (e.g., difficult falling asleep or frequent waking at night, even to go to the bathroom; 13.7 %), with 6.0 % diagnosed with sleep apnea; (2) evening edema, restless leg syndrome, varicose veins, or poor peripheral circulation (3.0 % diagnosed with restless leg syndrome); (3) mild cognitive difficulties (thinking and memory, general malaise, or “difficulty getting started,” or ADD/ADHD; 14.4 %); (4) psychological symptoms (e.g., anxiety, depression, or mood shifts; 14.9 %); (5) frequent headache or migraines (16.3 %); (6) gastrointestinal upset (e.g., constipation, abdominal cramps, nausea, irritable bowel, GERD or acid reflux; 16.6 %); or (7) occasional to frequent dizziness upon quick postural change, including standing (19.8 %). PE seems to mask sympathetic insufficiency upon standing. In pain patients, PE seems to underlie fibromyalgia. PE differentiates CRPS and vasovagal syncope, and a familial tendency has been observed, suggesting a genetic predisposition.
After treating for PE, more than half of the patients reported increased dizziness upon standing, especially in patients with CVD. As a result, when these patients are prescribed carvedilol, they are also prescribed low-dose midodrine, history dependent. We have also observed that correcting for this dynamic autonomic imbalance reduces the severity of the primary disease or disorder and, in some cases, eliminates symptoms all together.
Conclusion
The current working hypothesis is that sympathetic challenge (Valsalva or PC) PE is independent of the clinical state of the patient and may be treated independently of, and in addition to, the primary disease. This dynamic autonomic imbalance has been found to have clinical relevance. When corrected, patients report feeling better and their clinical state becomes stabilized as documented in longitudinal studies. These studies show that relieving PE reduces or relieves other autonomic dysfunctions and leads to stabilization of disease state (e.g., diabetes, hypertension, cardiomyopathy, and hypothyroidism).
PE seems to explain many patients with vague diffuse symptoms. In most cases where PE has been resolved and assuming no end-organ effects, patients have been weaned from pharmaceutical therapy in 15 months or less. Based on our clinical observations, it seems that the patients’ ANS may be retrained to function at a different “set point” and, if end-organ effects are also relieved, left to carry on independent of clinical support once PE is resolved [5].
Baroreceptor Reflex
The following are excerpts from a published article from the lab of Dr. S. Akselrod (an originator of P&S monitoring) [25]. Techniques to evaluate baroreflex sensitivity (BRS) are based on the analysis of BP or HR time series in the time or frequency domain. As Pagani et al. have documented, frequency domain analysis of BP or HR time series result in the same spectral content [26], namely, both provide very low frequency (VLF), low frequency (LF), and high frequency (HF) measures that cover the same frequency ranges: 0.003–0.04 Hz, 0.04–0.15 Hz, and 0.15–0.40 Hz, respectively [27, 28]. This should not be surprising due to the fact that both HR and BP time series originate from the same heartbeat interval (HBI) time series.
These approaches do not require injection of vasoactive substances, invasive arterial lines, nor do they rely on a complex experimental setup. Modern techniques for the estimation of spontaneous BRS are based on a variety of signal processing schemes and derive information on the baroreflex function from different perspectives. Thus, factors such as respiration (respiratory sinus arrhythmia) and other nonstationary agents may have different influences on the estimates provided by each of these approaches. Notwithstanding such individual specificity, however, it has been observed under both physiological and pathophysiological conditions that these techniques provide comparable information on BRS changes over time.
BP is characterized by continuous fluctuations, including fast changes lasting only a few seconds as well as slower and more prolonged variations, with a time constant of minutes or hours. Assessing the relative contribution of these different components to overall BP variance is now possible through a number of mathematical approaches, either in the time or in the frequency domain. Assessment of moment-by-moment changes in BP requires continuous, beat-by-beat, BP recordings. However, temporal information may also be derived from analysis of discontinuous BP tracings, such as those commonly performed in a clinical setting [29].
Just as with HRV, spectral analysis of btbBP is required to assess the forces driving BP variations. The low frequency component of beat-to-beat BP variations is considered an indication of baroreceptor reflex (BRR). This is the same LF region as for HRV, namely, 0.04–0.15 Hz [27, 28]. In other words, the same index of sympathetic activity underlies both HR variability and BP variability. Again, this should not be surprising, since both HR and BP time series are derived from the same HBI time series. In fact, given that the HR time series is derived from the RR interval of the EKG and that the R peaks of the EKG are much more well defined than the peaks of the BP time series, it may be argued that the spectral content from the HRV time series is more accurate than the spectral content from the btbBP time series. Either way, the LF region is still a mixed measure [30, 31] and requires a measure of respiratory activity to isolate P from S activity. What is still missing in this field to fully support the clinical applicability of the available observations on the prognostic value of circadian BP variations is a convincing demonstration that pharmacological correction of abnormal BP variability patterns is associated with an improved prognosis [32]. Although, more recently, another algorithm to determine BRS was developed.
Figure 14.5 [33] depicts the systolic BP (sBP) changes before, during, and after a 15 s Valsalva maneuver held near 40 mmHg. The shaded region is considered the BP recovery time (RecTime). BRS is determined as



Fig. 14.5
Example of heart rate (HR), systolic blood pressure (SBP), and diastolic blood pressure (DBP) profiles during the Valsalva maneuver (VM) in a healthy control subject. The part of phase 3 from which adrenergic baroreflex sensitivity (BRS) and BP recovery time (“RecTime” in the BRS equation) are derived is highlighted. “bpm” indicates beats per minute; 2_E, early phase 2 of the VM; and 2_L, late phase 2 of the VM. See text for details and BRS equation parameters: “A,” “B,” etc. (Adapted with permission from Schrezenmaier et al. [33])

The parameters are defined in Fig. 14.5. This approach benefits from the fact that the patient is holding their breath during the Valsalva maneuver and, therefore, effects of respiratory activity are minimized. However, in the average clinic, for the average patient, consistently establishing and maintaining a 40 mmHg Valsalva maneuver for 15 s is difficult, at best, and requires a sterile pack for the breathing tube. The additional technician and clinic time required to properly train the patient prolongs the test and lastly the training itself affects the results, if for nothing else causing the patient fatigue (especially, e.g.,, heart failure patients). Furthermore, forcing 15-s, 40 mmHg Valsalva maneuvers on high-risk patients (e.g., at risk for MI, stroke, retinal disease, or kidney disease) is not advisable and may preclude these patients from proper disease management.
Given that P&S monitoring accounts for respiratory activity and provides a specific measure of sympathetic activity, and given that sympathetic activity mediates BRR and thereby BRS, and given that (except in very extreme cases) the treatment for sympathetic dysfunction is the same as the treatment for BRR dysfunction, time-frequency analysis of HRV and RA provides comparable clinical information. Regarding BRR- and HRV-based measures of cardiac sympathetic innervation, Dr. Goldstein et al. have published five manuscripts [34–38], all stating that low-frequency (LF) power of HRV reflects baroreceptor reflex function not cardiac sympathetic innervation. Two of the articles are the same, the Cleveland Clinic Journal article [34] is a reprint with permission of a Heart Rhythm article [35]. These two articles reference only data from HRV-alone LF measures, not HRV measures corrected for respiratory activity. Also note that the article [36] is from the published abstract [37] presenting the same data by the same authors at the 21st International Symposium of the American Autonomic Society, Marco Island, Florida, 3–6 November 2010. Lastly, while the last article listed [38] includes a reference to [36] (and thereby [37]), the data presented do not include HRV-corrected data.
It is agreed, as Dr. Goldstein et al. state in the titles of these articles [34, 35, 38], that the HRV-alone measure of LF is not a measure of cardiac sympathetic innervation. Rather, Dr. Goldstein suggests that LF power reflects baroreflex function. Reading further, the first article [34, 35] while using a larger subject population measures sympathetic activity with HRV-alone or btbBP-alone technologies. The second article [38], a review article, while it specifically references P&S monitoring [39], many of the arguments made and all of the data presented are based on HRV-alone results, specifically LF power measures without respiratory activity analysis. Yes, it is agreed that LF power (not from P&S monitoring) does not reliably reflect sympathetic activity. These articles state that LF power is more reflective of BRS. While this may be true, it does not seem to address the relationship between P&S monitoring and BRS. Therefore, given earlier published statements [25], P&S monitoring provides a reliable and specific sympathetic measure that, by accounting for respiratory activity, provides more information regarding sympathetic activity, and thereby BRS, in a simple and more standard clinical setting [25], is suitable for primary care and prevention and helping to find the cases that require more expert intervention. Remembering that all of these methods are simply models of BRS (including the equation above), the patients for whom the differential between sympathetic and BRS function is important are those that would be referred to the more sophisticated autonomic labs.
The data set published in the other two manuscripts [36, 37] lead Dr. Goldstein to conclude [36]:
The ANSAR ANX 3.0 generates reports that include possible diagnoses and treatment options. Although the device correctly identified chronic autonomic nervous system dysfunction in most (but not all) patients with neurogenic orthostatic hypotension (OH), it is clear from our results that ANSAR reports often include erroneous diagnoses and consequently inappropriate treatment recommendations. By defining OH in terms of blood pressure changes between the seated position and 2 min upright, the ANSAR device failed to detect OH in patients who had unequivocal OH by generally accepted criteria. The ANSAR device also failed to identify OH as neurogenic in all patients who actually had neurogenic OH, due to the assumption that an increase in heart rate during orthostasis excludes neurogenic OH, whereas virtually all patients with neurogenic OH had at least some orthostatic increase in heart rate. Because of these errors the ANSAR device inappropriately recommended treatment with alpha lipoic acid for most of the patients in this study who had neurogenic OH.
The quotation begins with the statement that not all patients diagnosed with OH were properly identified as having autonomic dysfunction. There were only 33 patients enrolled in this study, and these patients were diagnosed with pure autonomic failure (N = 7), multiple system atrophy (N = 10), or Parkinson’s disease with (N = 3) or without (N = 5) orthostatic hypotension or were patients without autonomic failure or evidence of central neurodegeneration (N = 4) as one control group or normal volunteers (N = 4) as another control group. None of these Ns make for a statistically significant sample group, individually or collectively.
In the article’s Methods section [36], the authors state that the exact calculations were not available, when in fact they have been available to all since 1981 [39–44]. Also in the Methods section, they erroneously state that OH is diagnosed after 2 min of standing. In fact, the stand or head-up postural change (PC) portion of the autonomic assessment (equivalent to passive head-up tilt [45]) is programmed to be a minimum of five (5) minutes to meet the standards established by Ewing [46–48] and required by Medicare (CMS) [49]. By shortening the time frame over which the postural change response is measured, the response is artificially inflated resulting in a more normal-looking response. This is likely to account for the alleged failure to identify OH patients. These two errors would have easily been avoided if, in fact, ANSAR was permitted to properly train Dr. Goldstein’s lab when the device was delivered.
The allegation of a failure to properly identify patients with neurogenic OH based on at least some orthostatic increase in heart rate (HR) is also falsely represented as a result of a two minute (rather than 5 min) PC challenge. It is well known that HR increases due to orthostatic challenge. In fact, during the first 30 s of a PC challenge, HR increased and is then expected to return to resting levels. Therefore, there is always some increase in HR, as the article confirms, but not a normal increase. However, as with above, the inappropriate 2 min assessment (rather than 5 min) artificially inflates the average HR response, causing the erroneous results reported by Dr. Goldstein and his lab. As a result, Dr. Goldstein incorrectly leads to the conclusion that the ANSAR device inappropriately recommends treatment.
The final error in the quotation alpha-lipoic acid (ALA) is recommended as a treatment for neurogenic OH. This is a significant misrepresentation of the recommendations presented. Any time orthostatic dysfunction is indicated, a range of possible therapy options is presented, from proper daily hydration to mechanical intervention to vasopressors and more is offered for consideration, and it is made very clear that the choice of one of these possibilities is based on the physician’s knowledge of the patient’s medical history. Nowhere in this list of recommended therapies for OH is ALA included. Where ALA is included for consideration is in a separate paragraph referencing autonomic dysfunction in general, and then only as a supplement, an antioxidant selective for nerves as presented in referenced studies, including [50–52].
As indicated in the Methods section of Dr. Goldstein’s article, only four of the 33 subjects were supposedly normal. As Dr. Goldstein states at the end of the Results section, “For 29 of the 33 subjects, including 3 of 4 normal volunteers, alpha-lipoic acid was recommended as treatment.” Given the proper interpretation of the recommendation for ALS, this is absolutely correct for all of the patients. It is also not surprising for the normal patients, since ANSAR identifies autonomic dysfunction long before symptoms of orthostatic dysfunction.
Secondary Orthostatic Dysfunction
Orthostasis Is One of the Most Debilitating Symptoms of Diabetes [53]
Orthostasis and its subforms are a common comorbidity in diabetes [6, 7, 54, 55] and other chronic diseases such as hypertension [56–58], CHF [59], COPD [60], Parkinsonism [37, 61–63], multiple sclerosis [64, 65], atrial fibrillation [66], sleep [67], and pain [68–70]. Asymptomatic orthostatic hypotension (OH) is far more common and is an independent risk factor for mortality and CVD [7]. The prevalence of asymptomatic OH increases with age, the presence of hypertension or diabetes, and the use of antihypertensive or other medications. More frequent assessment of OH in clinical practice is recommended [71, 72]. The association of supine hypertension and OH has been found in about 5.5 % of hypertensive patients and in up to 50 % of patients with OH. Patients with hypertension and OH typically present with troublesome symptoms, end-organ damage, and difficulties in clinical management [41].
OH is a common problem among elderly patients, associated with significant morbidity and mortality. Acute OH is usually secondary to medication, fluid or blood loss, or adrenal insufficiency. Chronic OH is frequently due to alterations in the BP regulatory mechanisms from the cumulative effects of age or hypertension or other diseases effecting autonomic dysfunction [73]. OH may contribute to hypertension and subsequent antihypertension therapy may exacerbate dizziness, confounding management. In Parkinson’s disease, OH reflects sympathetic neurocirculatory failure from generalized sympathetic denervation [45]. Those with symptoms often benefit from a stepped approach with initial non-pharmacological interventions, including avoidance of potentially hypotensive medications and use of physical counter maneuvers. If these measures prove inadequate and the patient remains persistently symptomatic, various pharmacological interventions may be added, including mineralocorticoids (e.g., fludrocortisone), alpha-1 adrenergic agonists (e.g., midodrine), and nonsteroidal anti-inflammatory drugs. In the most severe cases, the acetylcholinesterase inhibitor, pyridostigmine, may be considered [74]; however, it is typically only administered by properly trained subspecialists. The goals of treatment are to improve symptoms and to make the patient as ambulatory as possible, rather than trying to achieve arbitrary BP goals. With proper evaluation and management, the occurrence of adverse events, including falls, fracture, functional decline, and myocardial ischemia, may be significantly reduced [57, 75].
These are excerpts from a manuscript originally accepted as an abstract entitled “Enhanced frequency domain analysis replaces older heart rate variability methods” and presented to the Fourth Annual Diabetes Technology Meeting, Philadelphia, PA, 28–30 October 2004 [76].
Background
From normal autonomic physiology, it is known that the autonomic response to stand starts with a decrease in parasympathetic activity. This decrease potentiates the sympathetic increase that follows. The sympathetic increase causes the vasoconstriction required to support the heart in maintaining normal brain perfusion with head-up posture (see Fig. 5.2). P&S monitoring allows proper dissection of the ANS and its responses to challenge to further diagnostics. For example, OH is common in diabetics. OH is a failure of the (alpha-)SNS, but it could also be (partially) caused by an overdrive of the PSNS. Either way, it does not seem to be an ANS structural deficit, but rather a functional deficit. Identifying this difference facilitates the diagnosis and therapy of OH as a consequence of a primary disease such as diabetes.
Methods
Two or more P&S function tests were performed on 389 adult diabetic patients. The average age of the cohort is 63.2 (range is from 25 to 96, see Table 14.1), with 161 females. The cohort includes 354 NIDDM (average age 63.5) patients and 35 IDDM patients (average age 61.1). P&S function tests are based on P&S monitoring. See Fig. 3.18 and Chap. 5 of this compendium for a complete methodology describing the Autonomic Assessment. From these data, the patient’s P&S responses were computed in response to clinical challenges and at rest. These patients demonstrated symptoms of orthostatic dysfunction or SW upon standing (associated with orthostatic dysfunction) and were analyzed. A decrease in the P&S monitoring parameter that represents sympathetic activity (LFa), in response to a postural change, indicates SW. Orthostatic dysfunction is defined physiologically as SW upon postural change. Clinically, OH is defined as a decrease in BP upon standing of a 20 mmHg systolic and 10 mmHg diastolic.
Table 14.1
OH/SW study population, average age data
Age range | N | Ave. age | Female |
---|---|---|---|
<31 | 2 | 26.5 | 1 |
31 to 40 | 15 | 35.9 | 7 |
41 to 50 | 43 | 45.6 | 21 |
51 to 60 | 84 | 56.0 | 30 |
61 to 70 | 79 | 66.3 | 35 |
71 to 80 | 113 | 75.0 | 53 |
>81 | 24 | 83.4 | 13 |
Results
Of the 389 subjects in the cohort, 170 (43.7 %) patients demonstrate clinical symptoms of orthostasis. A drop in LFa upon standing identified 284 (73 %) patients with SW (symptomatic or asymptomatic). According to their clinical notes, physicians diagnosed 18 % with OH. All patients diagnosed with OH demonstrated clinical symptoms. The differences are statistically significant: between SW and physician diagnosis (p < 0.001) and between SW and the combination of diagnosis and symptoms (p < 0.002). SW identified orthostatic risk more frequently than any decrease in BP (73 % vs. 56 %). SW identified orthostasis and more often than the clinical definition of OH (73 % vs. 16 %). These results are similar to those of Stoupakis et al. [77].
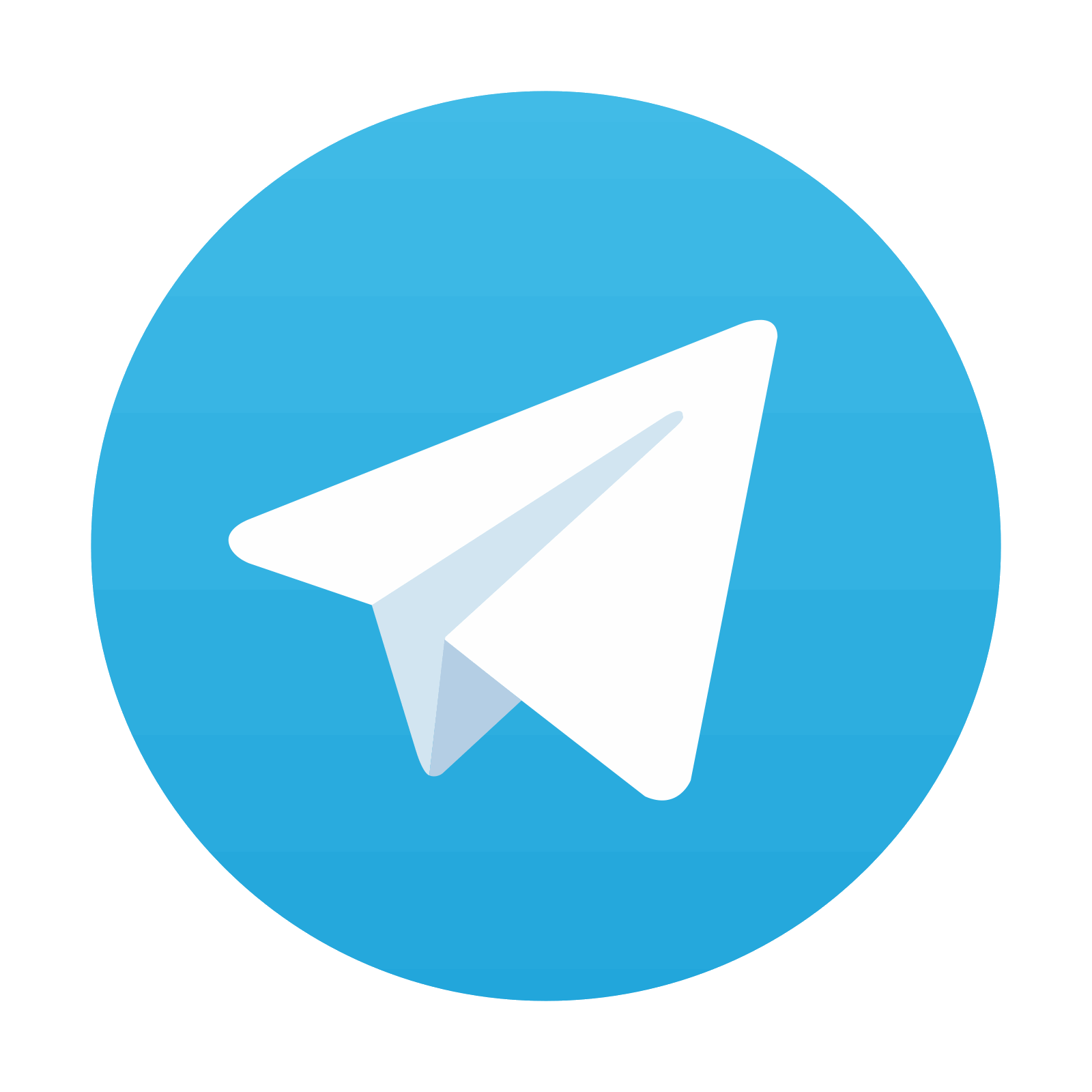
Stay updated, free articles. Join our Telegram channel
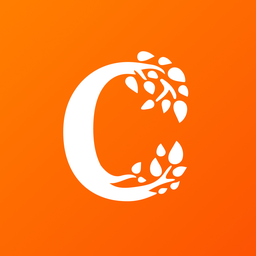
Full access? Get Clinical Tree
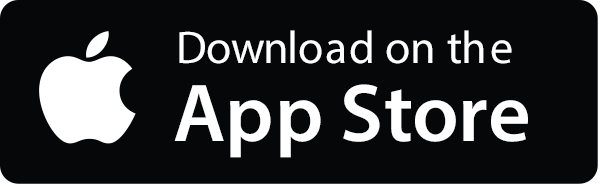
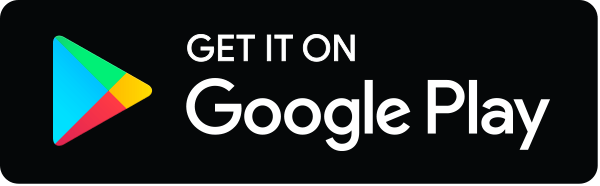