© Springer International Publishing Switzerland 2015
Dennis V. Cokkinos (ed.)Introduction to Translational Cardiovascular Research10.1007/978-3-319-08798-6_2929. Gene Therapy for the Heart
(1)
Laboratory of Cell and Gene Therapy, Biomedical Research Foundation of the Academy of Athens (IIBEAA), 4 Soranou Ephessiou Street, Athens, 115 27, Greece
(2)
Laboratory of Biology, University of Athens School of Medicine, 75 Mikras Asias Street, Athens, 11527, Greece
Abstract
Gene therapy refers to the use of DNA or of any other type of nucleic acid, as a pharmaceutical agent to treat a disease. This therapeutic approach was initially designed to treat several monogenic diseases, and is especially suited for the treatment of blood diseases such as hemoglobinopathies or immunodeficiencies. However, the advances in understanding the molecular basis of myocardial dysfunction, the identification and characterization of the properties and plasticity of several subpopulations of cardiac cells, combined with the development of increasingly efficient gene transfer technologies, has rendered heart failure another excellent candidate for gene-based therapies. Moreover, there is still a critical need to further explore novel therapeutic approaches in heart failure, and thus, gene therapy has emerged as a realistic alternative. Particularly, cardiovascular gene therapy has benefitted from recent advancements in vector technology and delivery modalities, and stem cell biology. This chapter reviews the available gene transfer technologies and the molecular features that constitute the main targets in the field of cardiac gene therapy, it presents the current status of gene therapy for the heart, and discusses how the knowledge gained from the initial clinical trials can be used to develop a safer and more efficient gene transfer approach. Since the first demonstration of the in vivo gene transfer into myocardium, there have been a series of advancements that have transformed gene therapy from an experimental tool to the threshold of becoming a viable clinical option.
Keywords
Gene therapyLentiviral vectorRetroviral integrationInsertional mutagenesisHematopoietic stem cells (HSCs)Cardiac stem cells (CSCs)β-adrenergic systemCalcium cycling proteinsHeart failureAbbreviations
AAV
Adeno-Associated Virus
ADA
Adenosine Deaminase
ADCY6
Adenylate Cyclase type 6
AdV
Adenovirus
β-AR
β-Adrenergic Receptor
CAR
Coxsackie-Adenovirus Receptor
CHF
Congestive Heart Failure
CMV
Cytomegalovirus
DRPs
DNase Resistant Particles
GRK
G protein-coupled Receptor Kinase
GTh
Gene Therapy
HIV
Human Immunodeficiency Virus
LTR
Long Terminal Repeat
LVAD
Left Ventricular Assist Device
MAR
Matrix Attachment Region elements
miRNAs
Micro Interfering RNAs
MLV
Murine Leukemia Virus
PAA
Polyamidoamine
PEI
Polyethylenimines
PIC
Pre-Integration Complex
PLN
Phospholamban
PP1
Protein Phosphatase 1
RNAi
RNA interfering
RSV
Rous Sarcoma Virus
SDF1
Stromal cell-Derived Factor-1
SERCA
Sarcoplasmic Reticulum Ca2+ ATPase
SIN
Self-inactivating
VSV
Vesicular Stomatitis Virus
VSV-G
Vesicular Stomatitis Virus envelope glycoprotein G
X-SCID
X-linked Severe Combined Immunodeficiency
29.1 Introduction
Gene therapy (GTh) refers to the use of DNA or of any other type of nucleic acid, as a pharmaceutical agent to treat a disease. The name derives from the idea that primarily DNA can be used to either substitute a deficient gene or replace a mutated gene within an individual’s cells. Thus, in gene therapy protocols, DNA encoding a therapeutic protein is packaged within a vector which is used to facilitate the entrance of DNA into the cells. Once inside the cell, in most types of viral vectors, the DNA integrates into the host genome and is expressed for life by the cell machinery, resulting in the production of the therapeutic protein, which eventually leads to the treatment of the disease.
Therapy by gene transfer in general, is not suitable for the treatment of complex disease phenotypes associated with multiple affected genes or for the multiple genetic variations that underlie complex disorders. Rather, gene therapy, with the exception of cancer and several other multifactorial disorders, is mostly applicable for monogenic disorders, such as the hereditary disorders caused by mutations in more than 1,800 gene loci [1]. Thus, it is conceivable that if sufficient correction or compensation can be achieved with gene transfer, monogenic disorders could be prevented and/or treated [1]. Therefore, this therapeutic approach was initially designed to treat several monogenic diseases and is especially suited for treatment of blood diseases such as hemoglobinopathies or immunodeficiencies.
However, the advances in understanding the molecular basis of myocardial dysfunction, the identification and characterization of the properties and plasticity of several subpopulations of cardiac cells, combined with the development of increasingly efficient gene transfer technologies, has rendered heart failure (HF) another excellent candidate for gene-based therapy.
The most important issue to resolve in a gene therapy trial is the adequate correction of the phenotype of the disease, by constructing the most efficient vector in terms of (a) gene delivery, (b) stability, (c) appropriate and tissue-specific transgene expression and (d) safety. Furthermore, the type of cell to be selected for correction, is of critical importance, and generally depends on the type of organ(s) and/or tissues that manifest the abnormal phenotype.
Three main types of gene therapy approaches are currently being employed:
(a)
In situ gene therapy in which the vector carrying the therapeutic genetic material is directly administered to the affected tissue, such as by an injection into a tumor nodule or organ [2]. This type of therapy applies for gene transfer to the heart.
(b)
Ex vivo gene therapy during which the patients’ cells are harvested and co-cultured in the laboratory in the presence of the therapeutic vector. The “corrected” cells with the new genetic material are then transplanted back to the patient from whom they were originally derived [2].
(c)
In vivo gene therapy is defined as the administration of the vector carrying the therapeutic genetic material directly to a live animal. The vector can be delivered by a variety of methods, such as intravenous injection or by other physical means of administration such as hypodermic injection, aerosol, or employing other routes [2].
29.2 Gene Therapy Vectors
Vectors constitute the vehicles or carriers that contain the therapeutic gene for delivery to the cells. Especially for the heart, the design must account for the tissue-specific and spatial patterns of the cardiovascular pathophysiological process, depending on whether it is a global process such as HF or a focal process such as nodal dysfunction. Therefore, the optimal vector format in HF must be able to effectively deliver and drive sustained transgene expression to guarantee long term improvement, as well as broad transduction efficiency to the majority of cardiomyocytes to ensure significant impact on ventricular function. In all cases, the designed vector should not induce cytotoxic effects and should be rescuable after injection in order to maximize the safety of the whole gene/cell therapy approach.
Gene delivery systems derive either from genetically engineered viruses or from non-viral formulations such as those manufactured by the use of nanotechnology [3]. Non-viral vectors include: (a) naked plasmid DNA, (b) liposomes, (c) DNA polymer-carrying particles and (d) oligonucleotides [4].
Several types of recombinant viruses are used as vehicles of the therapeutic genes in cardiac gene therapy. These include:
Retroviruses belonging to the subfamily of Oncoretroviridae e.g. Murine Leukemia Virus (MLV) or Lentiviridae e.g. Human Immunodeficiency Virus (HIV)
Adeno-Associated viruses
Adenoviruses
Each vector delivery system provides different specificity, exhibits different tropism and displays a distinct expression pattern at the molecular level. The appropriate choice of a delivery system will ensure a successful therapy. Moreover, the selection of the appropriate vector delivery method is critical for the proper implementation of the therapeutic strategy and for efficient transgene expression in the myocardium. Especially for cardiovascular disease, both the invasiveness of vector delivery method and the patient safety need to be critically assessed prior to initiating gene therapy trials. There are three ways of delivering the vectors to the myocardium and include (a) coronary artery and venous infusion, (b) direct intramyocardial injection and (c) pericardial delivery [4].
29.2.1 Retroviruses
29.2.1.1 Oncoretroviruses or Gamma-Retroviruses
Retroviruses are widely used as efficient tools for genetic manipulation of stem cells, mainly because of their ability to integrate into the host cell’s genome through the reverse transcription process during which the viral single-stranded (ss) RNA is converted to double-stranded (ds) DNA. Integration of the retroviral DNA genome into the host cell DNA is an essential step in the retrovirus replication cycle, permitting viral genomes to become permanently fixed as proviruses into the DNA of the host. During this process, the retroviral DNA is associated within a large complex with a subset of retroviral proteins known as the pre-integration complex (PIC). For oncoretroviruses, such as the murine leukemia virus (MLV), uncoating, DNA synthesis, and formation of the PIC occur at the same rate, both in non-dividing cells as well as in dividing cells, but integration fails to occur. However, during mitosis, the nuclear membrane disassembles, rendering the chromosomes accessible to the virus, suggesting that successful infection by oncoretroviruses such as MLV requires cell division [5].
Oncoretroviruses consist of an enveloped capsid that contains a plus (+) strand RNA genome ranging from 7 to 10 kb. Their tropism often includes hematopoietic stem cells. Oncoretroviral vectors present several advantages as they exhibit (a) efficient and stable gene transfer, transduction rates of up to 40 % of hematopoietic stem cells in non-human primates and (b) high fidelity gene transfer due to intact integration and absence of chromosomal rearrangements. However, their use in the clinic has been hampered by the development of T-cell acute lymphoblastic leukemia, due to integration of the viral genome in the second intron of the LMO-2 proto-oncogene in several patients during the gene therapy clinical trial for X-linked severe combined immunodeficiency (X-SCID) conducted in France [6]. Moreover, utilization of a gamma-retroviral vector led to genomic instability and myelodysplasia subsequent to EVI1 activation after gene therapy for chronic granulomatous disease [7]. It is therefore anticipated that oncoretroviral vectors will be gradually displaced in the field of hemopoietic gene therapy [8]. However, no studies have been conducted to date in the context of cardiac stem cells and/or cardiomyocytes and therefore, this field represents another challenge, due to the fact that there are basic issues that remain to be fully addressed regarding the level of myocyte regeneration.
29.2.1.2 Lentiviruses
Lentiviruses, display one major advantage compared to gamma-retroviruses as they do not require dissociation of the nuclear envelope in order to integrate their genome into the host’s genome, as it has been extensively documented, and therefore they can efficiently infect both dividing and non-dividing cells [9]. HIV in particular, has the capacity to cross the nuclear membrane of interphasic cells because the pre-integration complex contains a nuclear localization signal that allows transport through the nuclear pore complexes [10]. This represents a crucial aspect for genetically modifying tissues, especially those considered as the main potential cell targets of gene therapy, such as the brain, muscle, liver and the hematopoietic system, as they can transduce even non-dividing cells residing in the Go phase of the cell cycle.
Lentiviruses also consist of an enveloped capsid that contains a plus (+) strand RNA genome of approximately 10 kb. Wild-type HIV naturally infects cells of the hematopoietic system and specifically CD4+ T-cells. However, in the gene therapy context, HIV-based vectors are pseudotyped by alternative envelope glycoproteins such as the glycoprotein derived from vesicular stomatitis virus (VSV), namely the VSV-G envelope glycoprotein that confers significant tropism for many cells, including stem cells of the hematopoietic and the cardiac system. Pseudotyping with VSV-G presents an additional advantage, as it allows for the vector formulates to be concentrated by ultracentrifugation and therefore can lead to increased vector titers. Several human clinical trials utilizing lentiviral vectors have shown promise for various disorders including ADA-deficient SCID [11], β-thalassemia [12], Wiskott-Aldrich syndrome [13] and metachromatic leukodystrophy [14]. It is therefore clear that lentiviral vectors have outperformed oncoretroviral vectors in terms of safety and efficiency and represent an effective agent of gene transfer that can be also employed in the cardiac gene therapy field. However, although successful lentiviral transduction has been documented in cardiac micro-vascular endothelial cells [15], cardiomyocytes [16], cardiac stem cells and progenitors [17, 18], it seems that utilization of lentiviral vectors in the cardiac gene therapy field, is still far from reaching the clinic.
Despite the therapeutic effect that retroviruses have demonstrated, there is always the risk for insertional mutagenesis, i.e. activation of proto-oncogenes or down-regulation of tumour suppressor genes upon viral integration. While the possibility of insertional mutagenesis using replication-defective vectors has been discussed as theoretically possible [19], such risks had been originally estimated to be extremely low [20], based on the assumption that proviral integration into the genome was random [21].
29.2.1.3 Additional Safety Data on Retroviruses and Lentiviruses
With the readily accessible human genome sequence data, mapping studies of retroviral integration sites in cell lines, have uncovered non-random integration patterns, using wild-type HIV, HIV-derived, or murine leukemia virus (MLV)-derived vectors [22–26], while it was extensively documented that retroviral integration is actually not such a random process and that each virus presents a unique pattern of integration. Thus, MLV integrants are located predominantly around transcription start sites, while HIV integrants strongly favour transcription units and gene-dense regions of the genome. The basis for these preferences is unknown, and conceivably they may reflect interaction of the pre-integration complex with specific proteins or with specific DNA sequences or structures that are associated with transcription.
In order to make gene therapy based on retroviral vectors safer, the use of specific DNA elements called insulators, exhibiting the capacity to block enhancer activity and maintain the chromatin status of distinct chromosomal regions, was eventually introduced in the construction of vectors in order to (a) diminish variable expression and silencing of the transgene and (b) reduce the risk of insertional mutagenesis [27, 28]. This strategy combined with the deletion of the U3 region of the LTR in the self-inactivating (SIN) configuration of lentiviruses, has rendered over the past decade, lentiviral vectors as powerful tools in the fields of neuroscience, hematology, developmental biology, stem cell biology and transgenesis.
29.2.2 Adeno-Associated Viruses
Adeno-associated viruses (AAV) are members of the family Parvoviridae, and constitute non-pathogenic, non-enveloped viruses containing a 4.7 kb single-stranded DNA genome that encodes the structural proteins of the viral capsid, encoded by the cap gene and the non-structural proteins necessary for viral replication and assembly, encoded by the rep gene, flanked by short inverted terminal repeats. Their life cycle involves two phases, the replicative and the latent phase. In the productive phase, it co-infects the host cell only when a helper virus is present [29]. In the absence of a helper virus, AAV usually enters the latent phase, integrating into the human genome, commonly within a specific region of chromosome 19, although this has been observed only in cell lines. Its principal advantage for gene therapy is the poor inflammatory response to infected cells. As a therapeutic vector, AAV consists only of the inverted terminal repeats which are necessary for replication, packaging, and integration, while the viral coding sequences are entirely removed, rendering AAV vectors replication-deficient. The resulting recombinant vectors can efficiently deliver a transgene and safely mediate long-term gene expression in dividing and non-dividing cells of numerous tissues. AAV has the potential to facilitate long-term transgene expression in the absence of destructive T-cell responses, and such vectors have been generally proven safe. However, naturally occurring AAV variants are typically inefficient in infecting a number of stem cell types, particularly human embryonic stem cells [29].
There are 13 reported serotypes of AAV that display different tissue tropism, depending on the structure of the capsid protein. Serotypes AAV-1, 6, 8 and 9 have been shown to be the most cardiotropic. However, significant transduction in non-target tissues such as liver, skeletal muscle and lung has been documented [4]. In order to improve AAV cardiotropism, several methods have been introduced and novel viral capsid AAV libraries were constructed through DNA shuffling and chimeric strain production. This strategy simultaneously enhances tissue tropism and also helps AAV-based vectors to evade naturally occurring neutralizing antibodies [30–34]. Neutralizing antibodies to various AAV serotypes are present in approximately 20–80 % of the population, severely limiting the potential therapeutic use of AAV. Thus, the presence of such antibodies constitutes a major exclusion criterion in many AAV-based clinical trials [35]. There are numerous publications describing the efficient transduction of cardiac cells by AAV vectors [36, 37], and it appears that the AAVs constitute the most effective way for gene transfer in cardiac cells to date. Moreover, there are ongoing gene therapy clinical trials for HF in which gene transfer is mediated by AAV vectors.
Specifically, the first clinical trial of gene therapy was launched in the United States in 2007 [35, 38] and was based on gene delivery of the SERCA2 cDNA via a recombinant AAV1 (AAV1.SERCA2a) for calcium up-regulation in patients with advanced heart failure (CUPID). The study was a two-part, Phase 1/2 multicenter trial designed to evaluate the safety and the biological effects of gene transfer of the SERCA2a cDNA via the recombinant AAV1. In part 1, participants were administered a single intracoronary infusion of AAV1.SERCA2a in an open-label approach. A 12-month follow-up of these patients documented an acceptable safety pattern; moreover, improvement of cardiac function was detected in several patients. Overall, the results of the specific study suggested that gene transfer of AAV1.SERCA2a confers quantitative biological benefit. During the second part of the trial, 39 patients with advanced HF, received the AAV1-mediated SERCA2a transgene in one or three doses of DNase resistant particles (DRPs), i.e. low dose (6 × 1011 DRPs), middle dose (3 × 1012 DRPs), and high dose (1 × 1013 DRPs), or placebo [39]. Over a 1-year period, the cumulative recurrent cardiovascular events such as death, heart failure admission, left ventricular assist device (LVAD) insertion, cardiac transplantation, and myocardial infarction, increased in the placebo group but not in the low and middle-dose AAV1.SERCA2a groups which exhibited diminished recurrent cardiovascular events for the first 6 months. However, from months 6 to 12, these groups demonstrated events that were similar to placebo. The high-dose AAV1.SERCA2a group, continued to do significantly better than all groups at 12 months without any increase in adverse events, laboratory abnormalities, or arrhythmias [39]. Furthermore, the CUPID 2 Phase 2b trial (NCT01643330) is underway, designed to evaluate whether increasing SERCA2a activity via gene therapy improves clinical outcome [40]. Available data so far, indicate that calcium up-regulation by AAV1/SERCA2a gene therapy is safe and of potential benefit in advanced HF [40]. The promising results of the CUPID clinical trial, have prompted researchers to conduct two other clinical trials targeting SERCA2a, that will be enrolling patients soon. The first trial, which is conducted in the United Kingdom, includes patients with advanced heart failure who received LVAD at least 1 month prior to treatment. These patients will be treated either with AAV1.SERCA2a or saline. Simultaneously, a Phase 2, single-center, double-blind, randomized, placebo-controlled, parallel-group study will be conducted at the Institute de Cardiologie Pitié-Salpêtrière in Paris, France, with the primary objective of investigating the impact of AAV1.SERCA2a on cardiac remodelling parameters in patients with severe HF [4].
29.2.3 Adenoviruses
Human adenoviruses constitute another viral family used in gene therapy approaches, which are classified into 51 immunologically distinct serotypes divided into six subgroups, namely subgroups A–F. Adenoviruses are non-enveloped, double-stranded (ds) DNA viruses and contain large protein complexes that bind to CD46 or to coxsackie-adenovirus receptor (CAR), depending on the serotype for viral binding and cellular entry. Upon cellular entry via clathrin-mediated endocytosis, the dsDNA is transported to the nucleus across nuclear pores, allowing efficient transduction of both mitotic and non-mitotic cells. Their genome size ranges from approximately 30 to 35 kb and contains five segments that encode early gene products (E1a, E1b, E2a, E2b, E3, and E4), and five segments that encode late gene products (L1–L5). The E1, E2, and E4 gene products regulate transcription and translation of the late genes and therefore, are indispensable for viral replication [41]. Adenoviral vectors are among the most promising gene transfer vehicles for the in vivo treatment of a range of human diseases, e.g. cystic fibrosis and haemophilia, because of their ability to infect a wide spectrum of cell types, including quiescent cells. Adenoviral vectors present the following advantages: (a) they provide high efficiency of gene transfer because they are able to deliver many genome copies per target cell that typically translates into very high expression, (b) they can deliver relatively large therapeutic genes of approximately 25–30 kb in size especially with the latest generation vectors, and (c) they confer high fidelity of gene transfer because the vector genomes are genetically stable. However, although replication-deficient vectors based on adenoviruses can be produced easily and at high titres, two main disadvantages occur, i.e. (a) the transgene’s expression is transient and typically lasts 1–2 months in non-dividing cells, while is much shorter in dividing cells and (b) there is a significant induction of both cellular and humoral host immune response. First-generation adenoviral vectors may be applied where short-term expression and single dosing is desirable, such as cancer vaccine therapies. Initial enthusiasm toward the use of first-generation adenoviral vectors in gene replacement therapy has diminished, because not only they did fail to achieve sustained gene transfer, but also resulted in significant toxicity and death of an individual [41]. Furthermore, the pre-existing immunity against adenoviruses in patients, may result in low levels of transgene delivery and expression. Third-generation gutless or helper-dependent adenoviral vectors have attenuated immunogenic potential, but still a significant risk remains. Finally, CAR receptors are particularly prevalent in liver tissue, thus limiting adenoviral tissue specificity. Due to the aforementioned limiting factors, adenoviral mediated strategies are limited in cardiovascular gene therapy trials [42].
< div class='tao-gold-member'>
Only gold members can continue reading. Log In or Register a > to continue
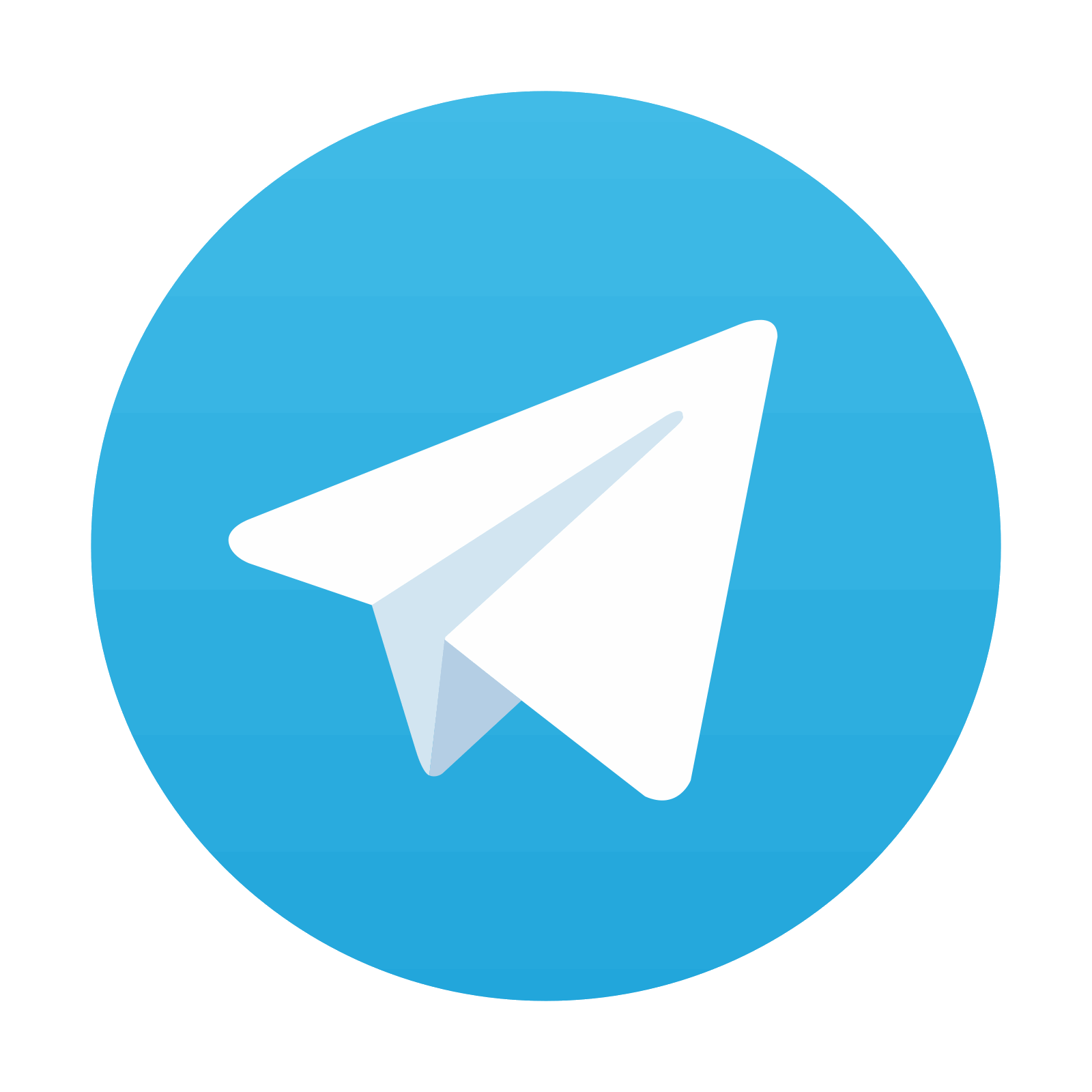
Stay updated, free articles. Join our Telegram channel
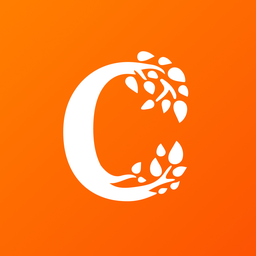
Full access? Get Clinical Tree
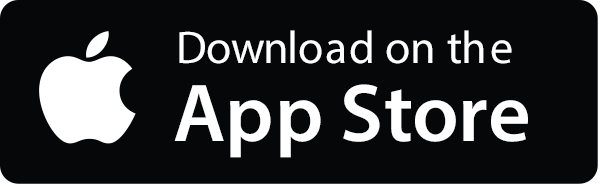
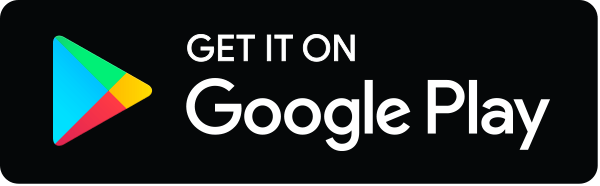