(1)
Division of Cardiovascular Medicine, University of Virginia, 1215 Lane Rd, Hospital Extension Building 4th floor, Charlottesville, VA 22908, USA
Keywords
Peripheral arterial diseaseCritical limb ischemiaAngiogenesisGene therapyCell therapyIntroduction
Peripheral arterial disease (PAD) is caused by atherosclerosis that results in narrowing and frequently complete occlusions of one or more arteries that supply the lower extremities. PAD affects ~8.5 million Americans at or over the age of 40 [1]. The two major clinical manifestations of PAD are intermittent claudication (IC) and critical limb ischemia (CLI). Intermittent claudication is defined by the presence of leg pain/cramping with walking that relieves with rest. CLI is defined as pain present at rest, with or without ischemic ulcers or gangrene, classified as Rutherford-Becker Class 4–6 or Fontaine Class III and IV. Patients with CLI are quite different than those with IC. The 1-year mortality rate in patients with CLI is approximately 25 %, and the overall amputation rate over 1 year is approximately 30 % [2]. While medical therapies to limit complications from atherosclerosis reduce general cardiovascular mortality in patients with CLI, at present there is no definitive medical therapy for CLI. Newer medications such as Praxilene (a metabolic enhancer and a 5-HT2 receptor antagonist) and Alprostadil (a prostaglandin E1 analogue) have been recently approved for use in Europe in patients with PAD to improve blood flow to the ischemic limb. However, mixed results from the clinic studies indicate that these drugs need further long-term evaluation to establish their role in PAD.
The primary clinical goal of treatment in critical limb ischemia is to relieve ischemic pain, promote wound healing, and reduce limb loss. Currently, these are commonly achieved with endovascular and/or surgical revascularization. However, many patients with CLI are not suitable candidates for revascularization based on their vessel anatomy, or the procedure is often unsuccessful due to graft failure and/or stent thrombosis or restenosis. Currently, there is no specific medical treatment directed at improving blood flow distal to vessel occlusion in CLI. To address this relative lack of specific medical therapy, current investigational approaches involve promotion of therapeutic angiogenesis in the limb distal to the occlusion site. Angiogenesis is the growth and proliferation of blood vessels from an existing vascular structure, with the potential to enhance tissue perfusion distal to a vessel occlusion. This chapter summarizes key approaches to therapeutic angiogenesis and clinical studies using genetic and cellular approaches to promote angiogenesis in CLI.
Angiogenesis
In the setting of an occlusion (s) in the artery(ies) that provides blood flow to the leg, perfusion becomes dependent on the growth of new blood vessels or neovascularization which is a physiological process that occurs as an adaptation to ischemia. The process of neovascularization can involve some or all of the processes of angiogenesis, arteriogenesis, and vasculogenesis [3–5].
Angiogenesis is the process of formation of new capillaries (vessels 8–12 μm in diameter) from preexisting blood vessels induced by the proliferation, differentiation, and migration of endothelial cells in response to stimuli such as hypoxia, ischemia, mechanical stretch, and inflammation. This process is regulated by a complex interaction of pro- and antiangiogenic growth factors, local tissue environment, and genetic factors (Fig. 44.1). Harnessing this physiological process using pharmacological and/or genetic modulation to enhance formation of new blood vessels distal to an arterial occlusion is known as therapeutic angiogenesis.


Fig. 44.1
Overview of angiogenesis . Signals from ischemic muscle result in release of pro-angiogenic transcription factors, growth factors, chemokines, and their receptors. These cumulatively results in basement membrane degradation, endothelial cell proliferation, migration, and maturation into new vessels, resulting in the process of angiogenesis. This process is under complex regulation by simultaneous effects of angiostatic factors, which prevent uncontrolled endothelial cell proliferation and help in the formation of more mature and functional vessels. However, impairing this balance in favor of more angiostatic factors results in impaired angiogenesis
Angiogenesis and Peripheral Arterial Disease
In PAD , tissue ischemia should lead to activation of hypoxia-inducible factor 1-alpha, a transcription factor that leads to downstream transcription of several pro-angiogenic growth factors and growth factor receptors, which cumulatively work to initiate endothelial cell sprouting, differentiation, and proliferation, thereby initiating the process of angiogenesis. Ischemia-induced angiogenesis can enhance tissue perfusion by providing a larger capillary surface area for blood flow in the microcirculation and thereby enhanced O2 delivery and also by reducing the peripheral resistance to blood flow.
It is highly likely that functional performance in patients with PAD is related to the amount of blood flow to leg muscle with exercise. Though measures such as the ankle-brachial blood pressure index (ABI) have not been able to predict functional capacity in PAD, we showed that in human subjects with PAD (intermittent claudication), lower capillary density in the ischemic tissue (calf muscle) correlates with worse functional performance [6]. Supervised exercise training has been shown to be the most effective treatment for patients with intermittent claudication to improve functional capacity and peak walking time. In patients with PAD who undergo supervised exercise training, angiogenesis in the ischemic calf muscles precedes changes in functional capacity. In addition, improvement in functional capacity was noted without an appreciable increase in blood flow [7], indicating a role of microcirculation in enhancing muscle performance independent of macrovascular measurable blood flow.
Approaches to Therapeutic Angiogenesis
The primary objective for therapeutic angiogenesis is to create a “pro-angiogenic” microenvironment in the ischemic tissue. Several different approaches have been under investigation to achieve this pro-angiogenic milieu. These involve direct injection of growth factors into ischemic tissue, gene therapy to promote pro-angiogenic growth factors in ischemic tissue, and stem/progenitor cell therapy to promote angiogenesis in the ischemic tissue.
Earlier studies investigated effects of direct injection of recombinant growth factors to ischemic tissue. However, direct protein therapy has significant limitations due to difficulty in routes of delivery, limited uptake by muscle cells, and short half -life of proteins. As such, gene therapy and cell therapy now are currently the focus of investigations in therapeutic angiogenesis.
Gene Therapy
Gene therapy involves insertion of genetic material to target cells or tissues to achieve sustained expression of the therapeutic protein of interest, with the aim to correct dysfunctional cellular processes underlying a disease. The origin of gene therapy dates back to 1960s, when the first evidence for uptake and expression of exogenous DNA in mammalian cells was obtained [8]. The advantages of gene therapy over protein delivery include prolonged and more controlled expression of the transgene products compared to transient expression and rapid degradation of proteins.
Modes of Delivery for Gene Therapy
Current techniques in delivering the gene of interest to cells/tissues include utilizing viral and nonviral vectors (Fig. 44.2). Viruses have the inherent capability to infect host cells and insert their genetic material. This property of viruses is utilized to achieve high transfection efficiency to deliver a target gene. The genetic material to be transferred is packaged into viral vectors with defective replication, but preserved ability to incorporate its genome into the host genome. This enables insertion of the transgene into cells without the vector virus replicating and infecting the host. The most common viral vectors used for gene therapy are different viral and nonviral gene delivery system including naked or plasmid DNA, which is inserted into cells using physical or chemical forces. Physical forces include electroporation, microinjection, particle bombardment, ultrasound bubbles, and hydrodynamic delivery. Chemical techniques include use of lipids or polymers capable of binding DNA and facilitating its transfer across the plasma membrane. Nonviral vectors have the advantage of low immunogenicity and therefore more flexibility for repeat dosing, but usually have low efficiencies and can lead to cellular toxicity. Viral vectors have the advantage of high transfection efficiency, but have high immunogenicity and have potential for insertion mutagenesis. Lentivirus (retrovirus that can infect both dividing and nondividing cells and can enter cells through intact cell membrane by membrane fusion) have the ability to provide high levels of gene expression. Adeno-associated viruses (AAV) are emerging as perhaps the most promising vector for gene therapy. AAV serotype 9 is well known to have selectivity for skeletal muscle, and we recently described the potential for even greater selectivity to ischemic skeletal muscle [9].


Fig. 44.2
Overview of gene therapy. Therapeutic transgene of interest is packaged into an expression cassette consisting of a promoter at the 5′ site and a polyadenylated sequence at 3′ site. The expression cassette is then inserted into viral vectors or nonviral vectors plasmids (packaged with liposomes). The vectors are then injected intramuscularly or intravenously Cellular uptake occurs by endocytosis, either receptor-mediated (viral vectors) or by liposome fusion in case of nonviral vectors. (In specific cases, alternate delivery using direct mechanical forces (electroporation) can cause direct cellular incorporation of plasmids.) The epichromosomal viral/plasmid DNA then undergoes transcription followed by translation, ultimately resulting in expression of the transgene protein, with resultant intended function of the protein
Gene Therapy in Critical Limb Ischemia
The primary goal of gene therapy in CLI is to promote therapeutic angiogenesis . Table 44.1 summarizes the different growth factors, transcription factors, and chemokines which have been tested till date to promote angiogenesis, in both clinical and preclinical settings. The most common pro-angiogenic growth factors or growth factor enhancers investigated in clinical trials include vascular endothelial growth factor (VEGF), fibroblast growth factor (FGF), hepatocyte growth factor (HGF), and HIF-1 alpha. Table 44.2 summarizes the key clinical trials of pro-angiogenic growth factors in patients with critical limb ischemia.
Table 44.1
Factors with angiogenic potential
Growth factors | VEGF A–E, PLGF, FGF-1, 2, 3, 5, angiopoietin-1 and 2, HGF, PDGF, GM-CSF, neurotrophin, IGF-1 and 2 |
Chemokines | MCP-1 |
Transcription factors | HIF-α, EGR-1, Prox-1 |
Table 44.2
Clinical trials of growth factors in CLI
Reference | Year | Study type | Gene | Vector | Route of delivery | Improvements in |
---|---|---|---|---|---|---|
Isner et al. [10] | 1996 | Phase I | VEGF | Plasmid | Intra-arterial | Collateral vessels and distal flow |
Baumgartner et al. [11] | 1998 | Phase I | VEGF165 | Plasmid | Intramuscular | Distal flow, ulcer healing |
Simovic et al. [12] | 2001 | Phase I | VEGF165 | Plasmid | Intramuscular | Symptom score, neurological exam, ABI, collaterals |
Kim et al. [13] | 2004 | Phase I | VEGF165 | Plasmid | Intramuscular | Collaterals |
Makinen et al. [14] | 2002 | Phase II | VEGF | Adenovirus | Intramuscular | Vascularity |
Kusumanto et al. [15] | 2006 | Phase II | VEGF165 | Plasmid | Intramuscular | Ulcer healing |
Hemodynamics | ||||||
Comerota et al. [16] | 2001 | Phase I | FGF-1 | Plasmid | Intramuscular | Pain |
Ulcer healing | ||||||
ABPI | ||||||
Transcutaneous oxygen pressure | ||||||
Nikol et al. [17] | 2008 | Phase II | FGF-1 | Plasmid | Intramuscular | Risk of amputation |
Belch et al. [18] | 2011 | Phase III | FGF-1 | Plasmid | Intramuscular | No benefit |
Morishita et al. [19] | 2004 | Phase I | HGF | Plasmid | Intramuscular | Pain, ABI, ulcer size |
Powell et al. [20] | 2008 | Phase II | HGF | Plasmid | Intramuscular | Transcutaneous oxygen pressure |
Powell et al. [21] | 2010 | Phase II | HGF | Plasmid | Intramuscular | Rest pain |
Shigematsu et al. [22] | 2010 | Phase III | HGF | Plasmid | Intramuscular | Rest pain, ulcer size, QOL |
Rajagopalan et al. [23] | 2007 | Phase I | HIF-1α | Adenovirus | Intramuscular | Rest pain, ulcer size |
Vascular Endothelial Growth Factor
The vascular endothelial growth factor family is the most well-characterized family of angiogenic growth factors, first identified in 1983 by Senger and Colleagues [24]. VEGF family consists of VEGF-A, VEGF-B, VEGF-C, VEGF-D, VEGF-E, and PLGF, which share a secretory signal sequence and binds to VEGF receptors 1, 2, and 3 with different affinities. VEGF-A has five different isoforms identified in humans, namely, VEGF-121, 145, 165,189, and 206, and VEGF 189 and 206 have extensive heparin-binding ability and are primarily matrix bound. VEGF 121 and 165 do not bind heparin and are primarily found in circulation [25, 26]. Knockout mice of all three VEGF receptors are lethal [27–30], indicating a coordinated role of signaling via all three VEGF receptors during developmental vasculogenesis. Recent reports have described that not all VEGF-A is angiogenic as indeed an antiangiogenic form has been identified in cancers and may be important in PAD [31].
Clinical Trials with VEGF Gene Therapy
Primary VEGF isoforms used in clinical trials to date has been VEGF 121 and VEGF 165, though approaches to increase all VEGF transcripts do exist. Several preclinical trials with VEGF gene therapy showed promising results in preclinical models of PAD [32–34].
Following the preclinical studies, Isner et al. did the first clinical trial using VEGF in humans in 1996 [10]. A patient was given phVEGF 165 intra-arterially to the distal popliteal artery using the hydrogel polymer coating of an angioplasty balloon. Digital subtraction angiography 4 weeks after gene therapy showed an increase in collateral vessels which persisted at 12 weeks follow-up. In addition, intra-arterial Doppler-flow studies also showed increased resting and maximum flows. In 1998, Baumgarther et al. administered phVGEF 165 by direct intramuscular injection into ischemic limbs of patients with CLI [35]. This study showed an increase in ankle-brachial index (ABI) and increased collateral vessels as evidenced by contrast angiography or MRA in the VEGF-treated patients. In a subsequent study done by Simvoic et al. [12] with phVEGF165 in patients with CLI, intramuscular injection of phVEGF165 resulted in increased ABI, reduced symptoms, and improved neurological sensory and motor functions, indicating a role of VEGF165 in chronic ischemic neuropathy. Kim et al. [13] injected plasmid DNA containing VEGF 165 gene (pCK) intramuscularly into patients with CLI and noted reduced pain, improved ulcer healing, improved ABI, and increased collaterals at 6 months follow-up.
The first phase II clinical study of VEGF 165 gene therapy was published by Makinen et al. in 2002 [14]. In a randomized, placebo-controlled, double-blinded study, patients received either ad-VEGF or VEGF-plasmid versus ringers lactate for controls. Injections were given via an intra-arterial catheter during PTA. Primary endpoint measured was vascularity, as assessed by digital subtraction angiography (DSA). DSA measured vascularity significantly increased compared to that in controls in both forms of VEGF treatment. Kusumanto and coworkers published a phase II study of VEGF gene therapy in patients with diabetes and CLI [15]. This was a double-blinded placebo-controlled study where VEGF gene carrying plasmid (phVEGF 165) or placebo (0.9 % NaCl) was administered intramuscularly to 54 diabetic patients with CLI. The primary endpoint studied was amputation rate at 100 days, and secondary endpoints were a 15 % or more increase in ABI/toe brachial index, clinical improvement, and safety. There was no improvement in the primary endpoint with VEGF therapy, but hemodynamic improvements and improvement in ulcer healing were noted.
Fibroblast Growth Factor
Fibroblast growth factors (FGF ) are a family of heparin-binding angiogenic growth factors with 22 structurally related proteins [36]. Several members of the FGF family have been shown to regulate developmental and various cellular pathways. FGF signaling also plays a significant role in angiogenesis. In particular, FGF-1 and FGF-2 have been the focus of therapies for promoting angiogenesis [17, 18, 37, 38].
Clinical Trials with FGF Gene Therapy
The first phase I clinical trial using FGF gene therapy was published in 2002 by Comerota et al. in 2002 [16]. In this study, a naked plasmid vector encoding FGF-1 (NV1FGF) was administered intramuscularly to the ischemic limbs of patients with CLI. The primary objective of this study was to evaluate the safety and tolerance of increasing and repeated (two) doses of NV1FGF. The secondary objectives were to determine the biologic activity of NV1FGF on hemodynamic and clinical parameters associated with improved perfusion. At 12 months follow-up, there NV-1FGF was found to be well tolerated. A significant improvement in ABI was noted posttreatment, and significant reduction in pain and aggregate ulcer size was noted, associated with an increased transcutaneous oxygen pressure compared with baseline pretreatment values. These encouraging results lead to the first phase II clinical trial with FGF in patients with CLI [17]. In this double-blinded, randomized placebo-controlled trial, investigators injected NV1FGF or placebo intramuscularly in ischemic limbs of patients with CLI. There was no significant improvement in ulcer healing, but the use of NV1FGF significantly reduced the risk of all amputations and major amputations compared to placebo. Following this, a phase III trial, TAMARIS, was published in 2011 [18]. In this study, 525 patients unsuitable for revascularization were randomly assigned to placebo or naked DNA plasmid with gene encoding FGF-1 (NV1FGF1) delivered intramuscularly. After 1-year follow-up, there was no difference in the primary endpoint to time to major amputation or death. Peripheral edema was the most commonly noted adverse effect.
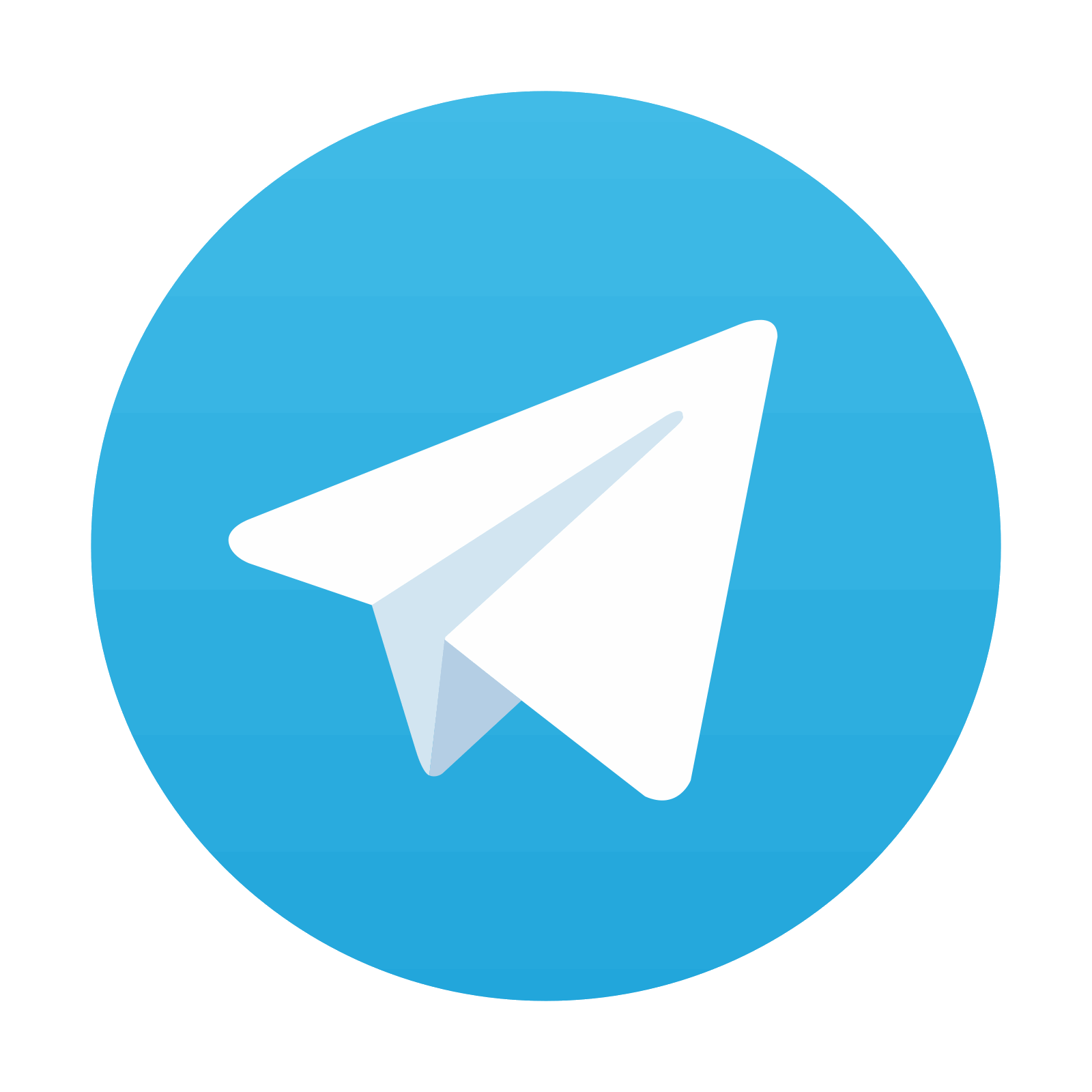
Stay updated, free articles. Join our Telegram channel
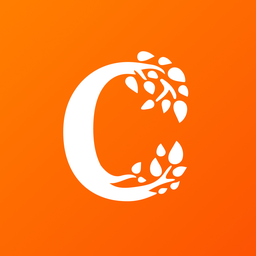
Full access? Get Clinical Tree
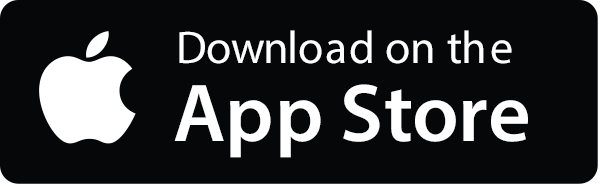
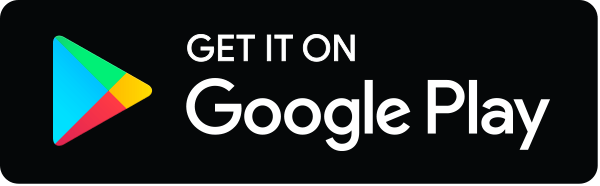
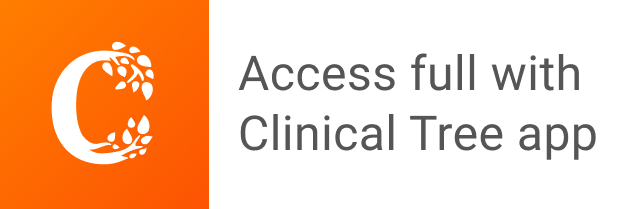