Since its birth in the late 1990s, strain echocardiography has continued to grow over the past 20 years. Currently, global longitudinal strain (GLS) has been standardized for clinical application as a robust diagnostic and prognostic marker with substantial incremental value over the traditional indicators of systolic function such as left ventricular ejection fraction (LVEF) in various diseases. However, several challenges remain to be addressed. More work is required to standardize strain values among vendors. The Strain Standardization Task Force led by the American Society of Echocardiography and European Association of Cardiovascular Imaging has helped to reduce the intervendor variability of GLS, , which has become a robust measure. The same cannot be said for regional strain, which shows test–retest variability ( Fig. 10.1 ) and continuing intervendor variability ( Fig. 10.2 ) in the transmural components of myocardial mechanics. These remain important barriers for clinical utilization. High dependency on image quality and lack of applicability for contrast-enhanced cardiac ultrasound images limits generalizability. Furthermore, lack of familiarity, the learning curve, and cognitive load that results from the display of strain curves and values can confound user interpretation and communication. Nevertheless, the recent introduction of a new current procedural terminology (CPT) code in the United States for reporting myocardial strain imaging has accelerated the clinical adoption of strain in clinical practice. Considering the renewed clinical interest, this chapter therefore attempts to develop a roadmap of opportunities and innovations that are likely to shape the future growth of strain echocardiography in the clinical and research settings.


Strain imaging as a biomarker for clinical trials
One of the imminent applications of strain imaging is its use as a surrogate endpoint for clinical trials. Although hard endpoints, such as mortality, are considered important in medical research, observing them requires a large sample size and a long follow-up. Traditionally, LVEF has been considered as the representative surrogate endpoint for reflecting change in cardiac systolic function. However, the reliability of GLS has grown with evidence of reproducibility when observers are properly trained, and clinical trials have begun employing strain as a reproducible and objective endpoint. For example, Ikonomidis et al studied the effect of interleukin-12 inhibition on cardiac function in patients with psoriasis using strain parameters as the primary outcome. They demonstrated that the improvement of GLS was significantly greater in the anti-interleukin drug arm compared with the tumor necrosis factor-alpha and cyclosporine arm. As such, many large cohort studies, such as Copenhagen Heart Study and ARIC Study, use speckle-tracking echocardiography for analysis of LV systolic function. , Interestingly, LVEF failed to show changes in systolic function in any arm. The Strain sUrveillance of Chemotherapy for improving Cardiovascular Outcomes (SUCCOUR) study is another interesting and unique study in which strain echocardiography is being compared in efficacy to conventional echocardiographic parameters. In the study, older adult patients who are undergoing chemotherapy using anthracyclines have been randomized to either a GLS-guided or an LVEF-guided arm ( Fig. 10.3 ). In the GLS-guided arm, a relative red uction of GLS by 12% in any follow-up echocardiography (3, 6, 9, and 12 months) is being considered as a threshold for initiating cardioprotective therapy, whereas protective therapy in the LVEF-guided arm is started in response to a conventional LVEF cutoff (a symptomatic drop of >5% of two-dimensional [2D] LVEF, or >10% asymptomatic drop to 2D LVEF <55%). Of 307 patients who were followed up over 1 year, significantly fewer patients developed CTRCD in the GLS-guided arm (9 [5.8%] vs 21 [13.7%], P = .022), although the primary endpoint of change in LVEF was similar (−3.0% vs −2.7%, P = .69).
Thus the ability of strain to identify subtle systolic dysfunction that cannot be appreciated by LVEF is driving its introduction into the mainstream in evaluation of systolic dysfunction especially in early stages of cardiovascular diseases. Collective evidence from randomized clinical trials may further help with formulation of recommendations for the use of strain in clinical practice guidelines. Furthermore, Aguilar and colleagues reported “archeological” strain echocardiography, which indicates assessing strain parameters from old-fashioned analogue archival echocardiographic images by digitalizing them. This technology may open up unique and interesting opportunities to use historical echocardiographic images to study the impact of subclinical LV dysfunction on outcome.
Three-dimensional strain imaging
Although two-dimensional (2D) strain imaging has been shown as a useful tool in clinical studies, there are several inherent limitations. First, the heart is a three-dimensional (3D) structure, and the direction of its movement (contraction and relaxation) occurs not only in the direction of ultrasound beam but also in the direction of the fiber orientation. Consequently, some portion of the myocardium also moves perpendicular to the ultrasound beam (through-plane phenomenon), and 2D speckle tracking may not be tracking the same myocardium throughout the cardiac cycle. Second, 2D strain imaging provides polar map (bull’s-eye map) of the strain values that is extrapolated to represent all segments of the heart. However, it is a reconstruction from only three standard views (apical, short axis, and parasternal views), which may overlook subtle regional abnormalities in areas that are not appreciated in these views. In addition, the orientation and positioning of the probe in acquisition of these three views can vary depending on the individual morphology of the heart. The heartbeat used for each of these three views is also different. These limitations can be overcome by using strain imaging from 3D echocardiography, which theoretically should be a better approach to study mechanical function of the heart. Although 3D echocardiography has had several limitations such as lower image quality and lower frame rates compared with 2D echocardiography, state-of-the-art technologies are making these limitations less significant. The latest 3D applications can scan the entire left ventricle over a single cardiac beat with good image quality with a volume rate of around 30/second, which is analogous to the temporal resolution of DICOM images that are often used for strain in postprocessing. Steps for standardizing the use of 3D strain and further head-to-head comparison with 2D strain in real-world settings will potentially allow verifying its applicability in clinical practice.
Principal strain, fiber orientation, and material properties
Although current strain imaging quantifies myocardial deformation in three independent directions (longitudinal, circumferential, and radial) or area of myocardial surface (area strain), the actual myocardium consists of three layers with different fiber and contraction orientations. Thus, none of the current strain directions corresponds to the specific orientation of physiologic myocardial contraction. Techniques such as principal strain analysis describes the multidimensional deformation of the myocardium and the direction along which it undergoes principal strains, which can simultaneously account for fiber direction and contraction orientations. Principal strain describes the direction of the largest deformation along which the stress is developed, which usually relates to the direction of myocardial fibers. The direction orthogonal to the “principal direction” is called the “secondary direction” ( Fig. 10.4 ). This approach may help in providing an integrated perspective of myocardial contraction rather than interpreting longitudinal, circumferential, and radial direction in isolation. Mangual et al applied principal strain analysis to healthy subjects and demonstrated that the principal strain lines are remarkably associated with the known orientation and activation sequence of the human muscle fibers ( Fig. 10.5 ). Furthermore, Pedrizzetti et al applied this approach to patients with hypertension showing that even though longitudinal and circumferential strains are similar, strain in a secondary direction could distinguish them from healthy subjects ( Fig. 10.6 ).


Besides the muscle fiber directions, myocardial material properties can be assessed using shear wave imaging. In this technique, a tiny segment of myocardium is displaced by acoustic radiation force generated by focused ultrasound, and the propagation of shear wave (the wave that spreads perpendicular to the direction of the displacement by shear stress) is observed using ultrafast echocardiography at a temporal resolution of thousands of frames per second ( Figs. 10.7 and 10.8 ). The shear waves propagate perpendicular to the primary wave direction, and the speed of propagation is determined by the fiber orientation (faster along than across the fiber direction) and the stiffness of the tissue (slower in soft tissues) ( Fig. 10.9 ). Using this technique, Lee et al assessed the myocardial fiber orientation of in vivo open-chest ovine hearts, whereas Pernot and colleagues , quantified diastolic stiffness of myocardium in open-chest ovine, healthy human adults, patients with hypertension, and patients with pediatric hypertrophic cardiomyopathy (see Fig. 10.9 ). Petrescu et al , further applied this technology without generating an artificial shear wave, instead using the shear wave naturally occurring from mitral and aortic valve closure ( Fig. 10.10 ). They demonstrated that the velocity of these naturally induced shear waves was higher (suggesting greater stiffness) in patients with cardiac amyloidosis and associated with age in healthy subjects, and that stiffness is associated with diastolic dysfunction and filling pressure ( Fig. 10.11 ).
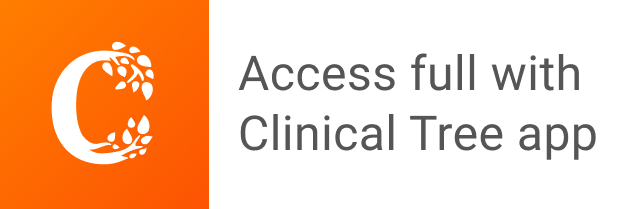