Chapter 2 Functional anatomy of the respiratory tract


This chapter is not a comprehensive account of respiratory anatomy but concentrates on those aspects that are most relevant to an understanding of function. The respiratory muscles are considered in Chapter 6.
Mouth, Nose and Pharynx
Breathing is normally possible through either the nose or the mouth, the two alternative air passages converging in the oro-pharynx. Nasal breathing is the norm and has two major advantages over mouth breathing – filtration of particulate matter by the vibrissae hairs and humidification of inspired gas. Humidification by the nose is highly efficient because the nasal septum and turbinates greatly increase the surface area of mucosa available for evaporation and produce turbulent flow so increasing contact between the mucosa and air. However, the nose may offer more resistance to air flow than the mouth, particularly when obstructed by polyps, adenoids or congestion of the nasal mucosa. Nasal resistance may make oral breathing obligatory and many children and adults breathe only or partly through their mouths at rest. With increasing levels of exercise in normal adults, the respiratory minute volume increases and at a level of about 35 l.min−1 the oral airway comes into play. Deflection of gas into either the nasal or the oral route is under voluntary control and accomplished with the soft palate, tongue and lips. These functions are best considered in relation to a midline sagittal section (Figure 2.1).
Part (C) shows the occlusion of the respiratory tract during a Valsalva manoeuvre. The airway is occluded at many sites: the lips are closed, the tongue is in contact with the hard palate anteriorly, the palatopharyngeal sphincter is tightly closed, the epiglottis is in contact with the posterior pharyngeal wall, and the vocal folds are closed so becoming visible in the midline in the figure.
Upper airway cross-sectional areas can be estimated from conventional radiographs, magnetic resonance imaging (MRI) as in Figure 2.1, or acoustic pharyngometry. In the latter technique, a single sound pulse of 100 μs duration is generated within the apparatus and passes along the airway of the subject. Recording of the timing and frequency of sound waves reflected back from the airway allows calculation of cross-sectional area which is then presented as a function of the distance travelled along the airway1 (Figure 2.2). Acoustic pharyngometry measurements correlate well with MRI scans of the airway,2 and the technique is now sufficiently developed for use in clinical situations with real-time results. For example, acoustic pharyngometry has been used following the placement of a tracheal tube to differentiate between oesophageal and tracheal intubation,3 and to estimate airway size in patients with sleep disordered breathing (Chapter 16).4
The Larynx
Speech.5 Phonation, the laryngeal component of speech, requires a combination of changes in position, tension and mass of the vocal folds (cords). Rotation of the arytenoid cartilages by the posterior cricoarytenoid muscles opens the vocal folds, while contraction of the lateral cricoarytenoid and oblique arytenoid muscles opposes this. With the vocal folds almost closed, the respiratory muscles generate a positive pressure of 5–35 cmH2O which may then be released by slight opening of the vocal folds to produce sound waves. The cricothyroid muscle tilts the cricoid and arytenoid cartilages backwards and also moves them posteriorly in relation to the thyroid cartilage. This produces up to 50% elongation and therefore tensioning of the vocal folds, an action opposed by the thyroarytenoid muscles, which draw the arytenoid cartilages forwards toward the thyroid and so shorten and relax the vocal folds. Tensioning of the cords results in both transverse and longitudinal resonance of the vocal fold allowing the formation of complex sound waves. The deeper fibres of the thyroarytenoids comprise the vocales muscles, which exert fine control over pitch of the voice by slight variations in both the tension and mass of the vocal folds. A more dramatic example of the effect of vocal fold mass on voice production occurs with inflammation of the laryngeal mucosa and the resulting hoarse voice or complete inability to phonate.
Effort closure. Tighter occlusion of the larynx, known as effort closure, is required for making expulsive efforts. It is also needed to lock the thoracic cage and so to secure the origin of the muscles of the upper arm arising from the rib cage, thus increasing the power which can be transmitted to the arm. In addition to simple apposition of the vocal folds described above, the aryepiglottic muscles and their continuation, the oblique and transverse arytenoids, act as a powerful sphincter capable of closing the inlet of the larynx, by bringing the aryepiglottic folds tightly together. The full process enables the larynx to withstand the highest pressures which can be generated in the thorax, usually at least 12 kPa (120 cmH2O) and often more. Sudden release of the obstruction is essential for effective coughing, when the linear velocity of air through the larynx is said to approach the speed of sound.
Laryngeal muscles are involved in controlling airways resistance, particularly during expiration, and this aspect of vocal fold function is described in Chapter 6.
The Tracheobronchial Tree
An accurate and complete model of the branching pattern of the human bronchial tree remains elusive, though several different models have been described.6 The most useful and widely accepted approach remains that of Weibel7 who numbered successive generations of air passages from the trachea (generation 0) down to alveolar sacs (generation 23). This ‘regular dichotomy’ model assumes that each bronchus regularly divides into two approximately equal size daughter bronchi. As a rough approximation it may therefore be assumed that the number of passages in each generation is double that in the previous generation, and the number of air passages in each generation is approximately indicated by the number 2 raised to the power of the generation number. This formula indicates one trachea, two main bronchi, four lobar bronchi, sixteen segmental bronchi, etc. However, this mathematical relationship is unlikely to be true in practice where bronchus length is variable, pairs of daughter bronchi are often unequal in size, and trifurcations may occur.
Work using computerised tomography to reconstruct, in three dimensions, the branching pattern of the airways has shown that a regular dichotomy system does occur for at least the first six generations.8 Beyond this point, the same study demonstrated trifurcation of some bronchi, and airways that terminated at generation 8. Table 2.1 traces the characteristics of progressive generations of airways in the respiratory tract.
Main, Lobar and Segmental Bronchi (Generations 1–4)
The trachea bifurcates asymmetrically, with the right bronchus being wider and making a smaller angle with the long axis of the trachea. Foreign bodies therefore tend to enter the right bronchus in preference to the left. Main, lobar and segmental bronchi have firm cartilaginous support in their walls, U-shaped in the main bronchi, but in the form of irregularly shaped and helical plates lower down with bronchial muscle between. Bronchi in this group (down to generation 4) are sufficiently regular to be individually named (Figure 2.3). Total cross-sectional area of the respiratory tract is minimal at the third generation (Figure 2.4).
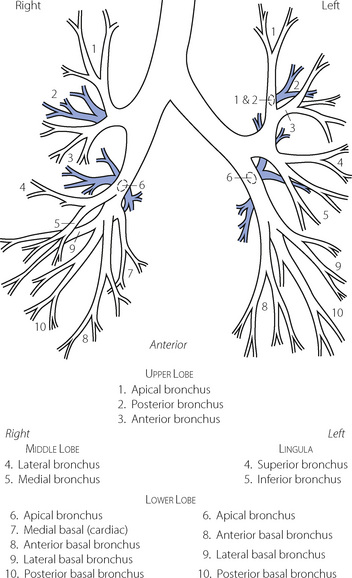
Fig. 2.3 Named branches of the tracheobronchial tree, viewed from the front.
(Reproduced by permission of the editors of Thorax.)
These bronchi are subjected to the full effect of changes in intrathoracic pressure and will collapse when the intrathoracic pressure exceeds the intraluminar pressure by about 5 kPa (50 cmH2O). This occurs in the larger bronchi during a forced expiration so limiting peak expiratory flow rate (see Figure 4.7).
Small Bronchi (Generations 5–11)
The small bronchi extend through about seven generations with their diameter progressively falling from 3.5 to 1 mm. Down to the level of the smallest true bronchi, air passages lie in close proximity to branches of the pulmonary artery in a sheath containing pulmonary lymphatics, which can be distended with oedema fluid giving rise to the characteristic ‘cuffing’ that is responsible for the earliest radiographic changes in pulmonary oedema. Because these air passages are not directly attached to the lung parenchyma they are not subject to direct traction and rely for their patency on cartilage within their walls and on the transmural pressure gradient, which is normally positive from lumen to intrathoracic space. In the normal subject this pressure gradient is seldom reversed and, even during a forced expiration, the intraluminar pressure in the small bronchi rapidly rises to more than 80% of the alveolar pressure, which is more than the extramural (intrathoracic) pressure.