Authors (publication year)
Procedure
Number of patients
Study design
Timing
Acute lung injury fluid volume
No acute lung injury fluid volume
P value
Zeldin et al. (1984) [4]
Pneumonectomy
25
Retrospective
24 h postoperative infusion
37 mL/kg
27 mL/kg
<0.05
Parquin et al. (1996) [14]
Pneumonectomy
146
Prospective (observational)
Intraoperative
≥2.0 L
<2.0 L
<0.01
Licker et al. (2003) [9]
Pulmonary resection
879
Retrospective
Intraoperative
9.1 mL/kg/h
7.2 mL/kg/h
0.023
Cumulated intra- and 24 h postoperative
2.6 mL/kg/h
2.0 mL/kg/h
0.003
Fluid balance 24 h postoperation
2.0 L
1.52 L
0.026
Fernández-Pérez et al. (2006) [16]
Pneumonectomy
170
Retrospective
Intraoperative
2.2 L
1.3 L
0.001
Alam et al. (2007) [15]
Pulmonary resection
1428
Retrospective
Intraoperative and 12 h postoperative
2.775 L
2.5 L
<0.05
Marret et al. (2010) [12]
Pneumonectomy
1200
Retrospective
Intraoperative
3.8 L
2.5 L
<0.0001
Mizuno et al. (2012) [18]
Pulmonary resection (pulmonary fibrosis patients)
52
Retrospective
Intraoperative total volume
7.71 mL/kg/h
10.3 mL/kg/h
0.049
Intraoperative fluid balance
4.99 mL/kg/h
8.00 mL/kg/h
0.035
Casado et al. (2010) [6]
Esophagectomy
45
Retrospective
Intraoperative fluid balance
5.415 L
4.174 L
0.01
Fluid balance 5 day postoperatively
7.873 L
5.928 L
0.03
Tandon et al. (2001) [19]
Esophagectomy
168
Retrospective
Intraoperative
5.0 L
4.4 L
<0.027
].
The incremental volume of fluid required to increase the risk of ALI is not large. It is evident from the study by Licker et al. [9] that there may be a small margin between a more “liberal” strategy (the volume of fluid administered that is associated with ALI) and a “conservative” approach (the volume of administered fluid not associated with ALI) [20]: while there was a significant difference in outcomes between patients receiving larger volumes of intraoperative fluid (9.1 v 7.2 ml/kg/h), higher positive fluid balance in the 24 h following surgery (2.0 v 1.52 L), and higher accumulated intra- and postoperative fluid volume (2.6 v 2.0 L), the differences are not great. Furthermore, a “dose-dependent” relationship between perioperative fluid administration and ALI was demonstrated by Alam et al. [15], who found that for every 500 mL of perioperative fluid administration, there was a significant increase in the rate of primary lung injury (OR 1.2 (1–1.4), p = 0.02).
5.3 Pathophysiology
A “multiple-hit hypothesis” for lung injury is well described for ARDS [21]. It describes a number of pathophysiological insults, which, in isolation, may not result in lung injury, however, when accumulated result in the clinical syndrome of ALI or ARDS. The “multiple-hit hypothesis” is likely to also be relevant in perioperative ALI. The “first hit” is an activation of the systemic inflammatory response by surgical trauma, manipulation, or atelectasis [22], which subclinically injures the lung, rendering it more susceptible to subsequent insults. The successive hits then damage the already vulnerable alveolar-capillary membrane, leading to overt ALI or ARDS. The putative second hit may be a variety of known risk factors for postoperative ALI such as FFP administration [13], mediastinal lymphatic damage [23], non-protective ventilation strategies [16], and oxygen toxicity [24].
This multiple-hit model for perioperative ALI is supported by a rodent model, which used intratracheal lipopolysaccharide to mimic sepsis-induced lung injury. A small lung injury was observed with either OLV and pneumonectomy or lipopolysaccharide alone, but an exaggerated injury was triggered when OLV, pneumonectomy, and lipopolysaccharide were combined in one animal [25]. This suggests that the lung is “primed” by the initial insult, and then a subsequent insult will potentially result in a more severe, clinically evident manifestation.
5.3.1 Revised Starling Equation and the Endothelial Glycocalyx
For generations, the axiom guiding transcapillary fluid behavior was Starling’s model, first proposed in 1896 [26]. The model expresses fluid flux as a balance between opposing hydrostatic and oncotic pressures. Along the length of the capillary filtration is favored at the arteriolar end and reabsorption at the venular end.
However, in vitro and in vivo deviations from the classic Starling principle have been noted [27], such as absence of the venous reabsorption [28] and lymphatic flow [29] required to prevent interstitial edema, and lack of importance of the interstitial colloid osmotic pressure in determining transendothelial fluid balance [30]. This led to further investigation into non-Starling mechanisms of barrier regulation involving the endothelial glycocalyx layer (EGL) [31].
Danielli first proposed the existence of the EGL in 1942 [32]. It is a dynamic, fragile, and complex layer of membrane-bound macromolecules at the luminal surface of the vascular endothelium [31]. The composition and thickness of the glycocalyx change constantly, as it is continually sheared by plasma flow and replaced [33]. Its components have a net negative charge and therefore repel negatively charged molecules and blood cells [34].
A primary function of EGL is to regulate and influence vascular permeability [35]. Together with circulating substances, it forms a barrier that prevents circulating cells and macromolecules from entering the interstitium. In contrast to the original Starling model, which explained regulation of fluid balance occurring across the entire endothelial cell, a revised model has been proposed whereby the hydrostatic and osmotic forces act only across the EGL surface layer on the luminal aspect of the endothelium [30]. These forces reach equilibrium very quickly, resulting in a much lower fluid flux than predicted by the traditional Starling equation (see Fig. 5.1).


Fig. 5.1
The glycocalyx model for fluid exchange between the intravascular (c) and interstitial (i) spaces. The various components of the endothelial glycocalyx layer (EGL) and revised Starling’s forces are shown. In steady state, net filtration into the interstitium occurs and is subsequently removed by the lymphatic system. EC endothelial capillary cells, π oncotic pressure, P hydrostatic pressure, g subglycocalyx space (Reproduced with permission from: Ashes and Slinger [3])
The EGL has other functions. It regulates blood cell-endothelial interaction by its negative charge and via specific adhesion molecules for leukocytes and platelets. These are normally hidden deep within the glycocalyx structure, but become exposed following damage to the EGL [35]. It also protects the vascular endothelium from shear stress and oxidative damage, via nitric oxide-induced vasodilation [36] and scavenging of oxygen free radicals [34].
The EGL may be injured by inflammatory cytokines [37], surgical trauma, and ischemia-reperfusion [38]. Hypervolemia damages the EGL, both by dilution of plasma proteins and via release of atrial natriuretic peptide, which strips the EGL [38]. Loss of the intact EGL causes increased vascular permeability and fluid extravasation. Loss of plasma proteins further compounds this. Leukocyte adhesion molecules are exposed, promoting cellular adhesion, migration, and further inflammation [39]. This vicious cycle of increased permeability, extravasation, and inflammation leads to pulmonary edema, as is observed in ALI.
Several empiric strategies, based on animal experiments, have been proposed to protect the EGL, including avoiding hypervolemia, albumin infusion [40], corticosteroids [41], antithrombin III [42], and direct inhibitors of inflammatory cytokines [43]. Volatile anesthetic agents, when compared with propofol, have been associated with less local release of inflammatory mediators [44, 45] and less glycocalyx destruction [46] (see Fig. 5.2).


Fig. 5.2
Electron microscopic views of hearts stained to reveal the glycocalyx. (a) An intact glycocalyx after 25 min. Of nonischemic perfusion. (b) A residual endothelial glycocalyx after 20 min of warm ischemia and 10 min consecutive reperfusion. (c) The glycocalyx after pretreatment with 1MAC of sevoflurane followed by 20 min of warm no-flow ischemia and 10 min reperfusion (Reproduced with permission from: Chappell et al. [46])
5.3.2 Pulmonary Endothelial Damage
The alveolar endothelium also plays a role in the regulation of pulmonary interstitial fluid balance. Fluid transport across the endothelium may occur via tight junctions, breaks in tight junctions and vesicular transport [47]. Leaky junctions are associated with cell death and allow passage of larger molecules. Epithelial sodium channels (ENaCs) are able to enhance the clearance of alveolar fluid [47], and they may be stimulated by beta-adrenergic agonists [48].
Endothelial damage has therefore been implicated in the pathogenesis ALI after lung resection surgery. Endothelial injury maybe induced by activation of systemic and local inflammatory mediators, related to positive pressure ventilation; “volutrauma;” oxygen toxicity; ischemia-reperfusion injury; surgical trauma; and preexisting lung disease [1, 3]. Endothelial cell injury results in disruption of intercellular endothelial cell junctions, cytoskeleton contraction, and cell death, leading to increased permeability of the alveolar-capillary barrier and decreased lung compliance [1].
5.3.3 Lymphatics and RV Dysfunction
Although fluid overload is well recognized as a risk factor for ALI after thoracic surgery, there also appear to be other factors at play. ALI may still occur when very strict fluid-restrictive strategies are implemented [49].
Lung lymphatics play a key role in fluid clearance from the lung [47]. Capillary filtrate that enters the interstitium is drained by lymphatics, and when their capacity to drain fluid is exceeded, pulmonary edema will occur [3]. Although lymph flow can increase sevenfold in response to elevated interstitial pressure [50], in the perioperative setting, this capacity may be reduced. Surgical trauma related to lung resection surgery is thought to be an important factor influencing this [3]. Pulmonary lymphatic drainage is not symmetrical: the drainage of the right lung is essentially ipsilateral (>90 %), whereas the left lung has a significant contralateral contribution (>55 %) [51]. Therefore, right pneumonectomy confers a significantly higher risk of pulmonary edema in the left lung, as over half its lymphatic drainage will be lost, which has been demonstrated clinically [7, 17].
Lymphatic drainage may be further impaired by postoperative right ventricular (RV) dysfunction: the resultant elevation in central venous pressure will reduce the drainage capacity of the lymphatic system [52]. RV dysfunction is very common after lung resection surgery, particularly pneumonectomy [53], and is thought to relate to increased RV afterload and tachycardia [54, 55].
5.4 Risks of Restrictive Approach: Tissue Hypoperfusion and AKI
A restrictive approach to fluid management has been widely adopted to prevent ALI after thoracic surgery [47]. However, a restrictive fluid regimen may incur the risks associated with hypovolemia, which include impaired end-organ perfusion, in particular, acute kidney injury (AKI) [3, 47].
Recent data suggest the incidence of AKI after lung resection surgery is 5.9–6.8 % [56, 57]. It is associated with increased hospital length of stay [56] and cardiopulmonary complications [57]. A link to increased mortality has been inconsistent [56], although a recent study demonstrated a mortality of 19.8 % in those with AKI [57].
Many risk factors for AKI have been identified. Studies by Licker et al. [1] and Ishikawa et al. [56] each identified multiple factors associated with AKI after lung resection surgery. Preoperative patient characteristics include ASA class 3 or 4, FEV1, hypertension, peripheral vascular disease, preoperative renal dysfunction, and preoperative use of angiotensin II receptor antagonists. Intraoperatively, use of vasopressor, duration of anesthesia, use of colloids, and open procedures were implicated. The amount of fluid given intra- and postoperatively was not found to be associated with an increased incidence of AKI: Licker et al. [57] found that patients with and without AKI received similar volumes of fluid both intraoperatively (4.8 v 4.9 mL/kg/h) and on postoperative day 1 (1.1 v 1.1 mL/kg/h), respectively. Similarly, Ishikawa et al. [56] found similar volumes of fluid administered intraoperatively in patients with and without AKI (1450 v 1276 mL).
Maintenance of adequate perfusion pressure is an important factor in preventing AKI [3], especially in those at increased preoperative risk related to chronic kidney disease, hypertension, or peripheral vascular disease. Hypotension related to excessive anesthesia should be avoided, and depth of anesthesia monitoring may allow more accurate dose titration. Adequate perfusion pressure should be maintained through judicious use of vasopressors, and invasive hemodynamic monitors may provide valuable information to help guide therapy [3].
5.5 Esophagectomy
In esophagectomy, the traditional approach involved aggressive fluid resuscitation, due to postulated “third space” losses [3]. The third space, first described in 1961 in major abdominal surgery [58], is classically thought to be a fluid compartment anatomically and functionally separate to the intravascular space, not involved in the exchange of fluid between the vascular space and the interstitium [59]. However, the exact location of this hypothetical compartment, thought to be the gastrointestinal tract or traumatized tissues, has never been fully elucidated. Its existence has recently been challenged due to weak initial evidence, flawed methodology, and the emergence of new data measuring extracellular fluid volume in surgery and hemorrhage [60].
There is an association between fluid balance and postoperative complications after esophagectomy. A link between higher perioperative positive fluid balance and cardiorespiratory complications and death has been demonstrated [61]. Fluid restriction seems protective against respiratory complications following esophagectomy, both as a sole factor [62], and as part of a standardized multimodal regimen including thoracic epidural analgesia, early extubation, and modest fluid restriction [63]. Due to the systemic inflammatory state that occurs following major surgery, and the increased capillary permeability that ensues, irrational replacement of putative “third space” losses during esophagectomy will lead to fluid accumulation in the interstitial space and therefore pulmonary edema [3].
Fluid administration may adversely affect surgical outcomes. There is a growing pool of data suggesting that surgical outcomes [64, 65] including anastomotic complications [66, 67], following gastrointestinal surgery, may be improved with a restrictive fluid strategy or multimodal perioperative management protocol that includes fluid restriction. There is no specific evidence of anastomotic protection by a restrictive fluid regimen in esophageal resection; however, extrapolation of these findings suggests that there may be some additional benefit incurred by fluid restriction in esophagectomy, both improving surgical outcomes and reducing the risk of ALI.
There has been concern regarding use of vasopressors in esophagectomy, due to fear of anastomotic ischemia, a major cause of postoperative mortality. In a porcine model, norepinephrine, when used to treat hypotension caused by hemorrhage, has been associated with severe graft hypoperfusion [68]. However, a small human study found that epinephrine, used to treat hypotension caused by thoracic epidural bupivacaine, restored the resultant decrease in anastomotic blood flow [69]. Similarly, in another small human study, phenylephrine infusion was found to correct epidural bolus-induced reduction of blood flow at the anastomotic end of the newly formed gastric tube [70]. Therefore, it is likely that vasoactive agents, when used to counteract hypotension induced by general or neuraxial anesthesia, can be used without jeopardizing the viability of the surgical anastomosis.
5.6 Goal-Directed Approaches
Goal-directed therapy has been used with variable success in cardiac, vascular, orthopedic, and major abdominal surgery. Interest was generated by Shoemaker et al. [71], who demonstrated morbidity and mortality benefits when a goal-directed approach was applied to the perioperative care of high-risk surgical patients. Some subsequent studies have shown reduced risk of infective complications [72], AKI [73], cardiovascular complications [74], pneumonia, and hospital length of stay [73]. However, others have found no benefit, in abdominal aortic surgery [75] or colorectal surgery [76]. In fact, a goal-directed approach was associated with negative effects on hospital length of stay and readiness for discharge in a subset of aerobically fit colorectal surgical patients [76].
It has been suggested that using clinical assessment of cardiac preload to guide fluid therapy may reduce the risk of ALI after thoracic surgery [3, 47, 77]: by optimizing the volume of fluid infused, the risks of both fluid overload and ALI and hypovolemia and AKI may be reduced. However, it should be noted that studies of goal-directed therapy in non-thoracic surgery frequently feature more “aggressive” fluid resuscitation in the treatment arm, with patients undergoing goal-directed therapy receiving significantly more fluid than those in the control arms [78–80]. This is at odds to the traditional approach of fluid restriction in thoracic surgical patients already discussed.
Preload estimation is notoriously challenging. Commonly used pressure measurements, such as central venous pressure (CVP) and pulmonary artery occlusion pressure (PAOP), are indirect surrogates for LVEDV and as such are influenced by many other factors, including intrathoracic pressure variation (such as in positive pressure ventilation and OLV), open chest surgery, RV function, and cardiovascular compliance [77]. Most goal-directed strategies utilize monitoring of hemodynamic parameters that predict fluid responsiveness, defined as a significant increase in cardiac output with fluid loading, which theoretically allows maximization of cardiac performance and avoids unnecessary volume administration.
5.6.1 Cardiac Index Estimation
Many goal-directed protocols target cardiac index, which may be measured using a variety of modalities, including the pulmonary artery catheter, transpulmonary thermodilution (e.g., PiCCO monitor), pulse contour analysis (e.g., FloTrac-Vigileo system), and transesophageal Doppler measurement. Although use of esophageal Doppler is impractical in esophageal surgery, it has been used with good effect in lung resection surgery. Esophageal Doppler was able to detect a reduction in stroke volume index in lung resection surgery, despite unchanged heart rate and mean arterial pressure, and was used to guide hemodynamic support and fluid therapy [81].
5.6.2 Dynamic Variables: Stroke Volume Variation (SVV) and Pulse Pressure Variation (PVV)
SVV and PPV both use the heart-lung interaction, integrating the effects of preload, respiratory variation, and blood pressure to assess fluid responsiveness [3, 47]. There are some theoretical limitations to the use of these indices during thoracic surgery. Firstly, there has been concern regarding their validity during open chest conditions [82]. Secondly, due to the dependence of these measurements on respiratory variation, their accuracy also is dependent on tidal volume. At relatively large tidal volumes of 8–10 mL/kg during two lung ventilation (TLV), SVV ≥ 12 ml/kg and PPV ≥ 10 mL/kg correlate highly with fluid responsiveness [83]; however, ventilation with a lung protective strategy may not have the same correlation. Thirdly, the volume of shunted blood through the non-ventilated lung should not contribute to the generation of SVV and PVV, necessitating a lower threshold value during OLV than that used during TLV [3, 84]. In one study examining SVV during OLV, it was shown only to be acceptably predictive of volume responsiveness with tidal volumes >8 mL/kg, with a threshold for fluid responsiveness of 10.5 % [85]. At the upper limits of fluid responsiveness, small increases in cardiac output are associated with large increases in extravascular lung water. This is particularly a problem in conditions of increased endothelial capillary permeability (see Fig. 5.3) [84].
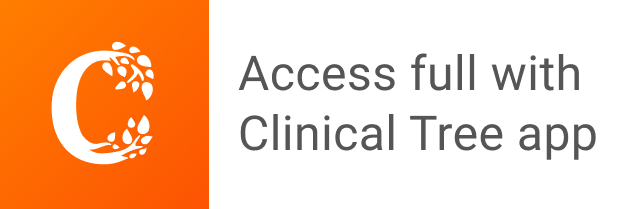