Fig. 16.1
A phase shift of the MR signal relative to static tissue is imparted to moving tissue (a, left) when a bipolar gradient is applied during signal acquisition (b, right). The phase shift can be thought of as a time shift between the signal from the static and moving spins. A bi-polar gradient is a gradient that has positive and negative components with the same area
PCMR imparts this phase shift by using a bipolar magnetic field gradient during the signal acquisition. A magnetic field gradient is a linear variation of the main magnetic field in a single direction. The gradient is controlled by a set of electromagnets separate from the main magnetic field. Gradients are characterized by the time they are on (t), the direction of the variation (x, y, or z) and the value representing the slope of the field versus distance (G). The gradient G z (t) is a gradient with a positive slope value of G in the z-direction for a time t. A bipolar gradient is a gradient that is on for the same amount of time (t) in the positive (G) and negative (−G) directions (i.e., first moment = 0), Fig. 16.1b. When a bipolar gradient is applied in the direction blood is flowing, the blood will have a phase shift proportional to its motion over the time the gradient is applied. This bipolar gradient has no effect on static tissue, but imparts a phase shift to moving blood that is proportional to its velocity.
If one ignores higher order motion terms such as acceleration, the imparted phase shift is proportional to the blood velocity to within a constant. By acquiring a phase image with a bipolar gradient (velocity-encoded image) and reference phase image (velocity-compensated image) and subtracting them, the majority of the background phase shift can be removed from the images. The value which relates the measured phase shift after subtraction to the velocity is called the velocity encoding (VENC) value, and is related to the strength and duration of the applied gradients. The formula for the relationship is shown in Eq. 16.1.
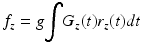
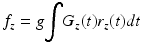
(16.1)
This is the generalized equation for phase shift. If Gz is a known bipolar gradient waveform (area under curve = 0), and we assume position of spins is: rz(t) = r0 + vzt, the velocity can be determined within a constant. Velocity encoded and non-encoded images are acquired and subtracted to remove much of the residual background phase constant. The velocity encoding value (VENC) is the proportionality constant between velocity and phase that takes into account gradient strength and durations.
An example PCMR image after subtraction along with a corresponding magnitude image from a transverse slice through the ascending aorta at peak systole is shown in Fig. 16.2. The intensity values in the phase images are directly proportional to the velocity of spins within the voxel in the direction of the velocity encoding on a pixel-by-pixel basis. In a PCMR image, mid-grey level represents static tissue, bright signal represents flow toward the head, and the dark signal represents flow toward the feet.
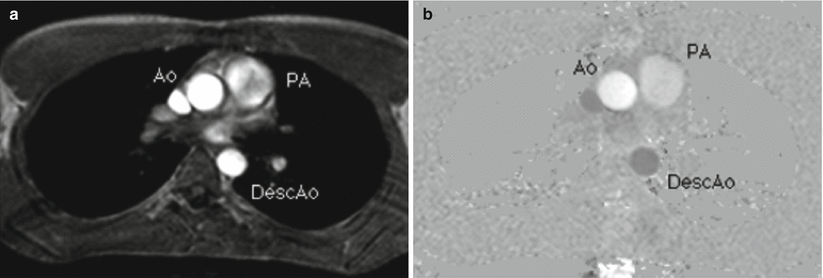
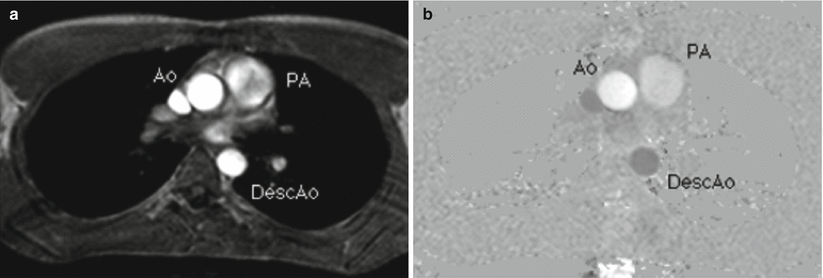
Fig. 16.2
Magnitude (left) and phase (right) images from a transverse slice through the chest just superior to the aortic valve at peak systole. Velocity is encoded in the foot-to-head direction. In the phase image the ascending aorta (Ao) has bright signal, indicating flow toward the head. The descending aorta (DescAo) is dark, indicating flow toward the feet. The signal intensity directly represents velocity on a pixel-by-pixel basis. The Pulmonary Artery (PA) velocity is lower as the area is higher in the slice location. A region of interest (ROI) is shown around the aorta to indicate the area over which to sum velocity measurements in order to get flow values
Implementation of PCMR
In the previous section, we presented an overview of how PCMR works to measure velocity. In the following section, we will present few details on the implementation of PCMR and how its implementation may affect clinical measurements.
PCMR Pulse Sequences
The majority of implementations of PCMR require the use a rapid, low flip angle, gradient echo sequence. The sequence employs a short repetition time (TR) and a short echo time (TE), which minimizes de-phasing due to the presence of complex flow and increases temporal resolution when cardiac gating is used [3]. The lower flip angle reduces radiofrequency energy deposition and keeps TE as short as possible. In many applications where flow measurements are made in or near the heart, respiratory compensation is required to reduce blurring and ghosting in the images. In order to reduce acquisition time and complete the acquisition in a breath hold, segmented acquisitions strategies are often employed in which several lines of k-space are acquired for each cardiac phase. The larger the number of k-space lines that are acquired per cardiac phase, the shorter the overall acquisition time (Fig. 16.3). The penalty for the shorter overall acquisition time is a longer temporal acquisition window (reducing the true temporal resolution). Variations on this pulse sequence include the use of echo-planar techniques [4], spiral readouts strategies [5], and steady state free procession (SSFP) [6].
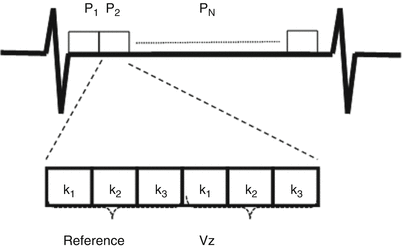
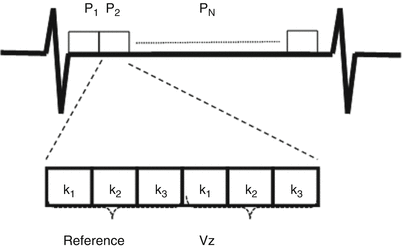
Fig. 16.3
Example of k-space segmentation in PCMR. In the diagram, 3 k-space lines (k1, k2, k3) are acquired per cardiac phase (P1…PN). Because reference (non-encoded) and velocity encoded (Vz) images are acquired, the temporal window is increased and effective temporal resolution in decreased. If more lines are acquired per cardiac phase temporal resolution will decrease (lower number of true cardiac phases), but the scan duration will decrease
Cardiac Gating and Temporal Resolution
In most clinical applications of PCMR, cardiac gating is required so that multiple images at equally spaced time points over the cardiac cycle can be generated and displayed. The gating will allow generation of time versus velocity or time versus flow curves over the cardiac cycle. Prospective cardiac gating means that when the R-wave is detected, a sequence is run over a specific time, usually ~90 % of the estimated cardiac cycle. When the next R-wave is detected, the sequence is run again with a new set of phase encoding values, and so on until the entire imaging sequence is complete. Retrospective cardiac gating acquires data continuously and keeps track of the position of the R-wave in relation to the k-space data [7]. When the acquisition is completed, data is binned into temporal phases in relation to the R-wave for reconstruction. The advantage of retrospective gating is that the entire cardiac cycle is reconstructed, prospective gating will miss ~10 % of end-diastole. Retrospective gating also allows for nearly arbitrary reconstruction of the number of phases, however, temporal smoothing occurs during reconstruction. The true temporal resolution of a PCMR scan can be determined by multiplying the TR by the number of segments acquired and by two (for velocity encoded and non-encoded image segments) (Fig. 16.3).
Spatial Resolution
The phase measurement from a voxel is the average phase over the entire voxel. If the pixel contains a mixture of static tissue and moving tissue, the velocity will reflect this average phase value. For flow measurements integrated over the entire vessel area this averaging has little effect on accuracy until there are less than approximately four pixels across the vessel diameter [8]. However, for estimating maximum velocity for pressure gradient calculations, or determining the velocity gradient near the wall for estimating wall shear stress, higher spatial resolution is required [9]. As with any MR sequence, increasing temporal resolution generally will increase acquisition time, decrease signal-to-noise ratio, or decrease spatial resolution.
Respiratory Compensation
As mentioned previously, in most cardiovascular applications, PCMR is executed in a breath hold to mitigate effects of respiratory motion. However, in some applications, either high spatial resolution is required, the patient cannot execute the breath hold, or a 3D volume needs to be covered. In these cases, the scan time exceeds the patient’s breath hold duration capacity. Multiple signal averages can be acquired, but this often produces unacceptable image quality results and long scan times. In these cases, a navigator echo respiratory gating scheme can be employed. The navigator echo is a localized excitation beam that produces one-dimensional, time-dependent images. The beam is usually positioned over the right hemi-diaphragm and monitors the respiratory position of the diaphragm. The beam is usually executed at the beginning of the cardiac cycle and a decision is made whether the diaphragm position is within a user-defined respiratory gating window. If so, the data is used for image reconstruction, if not it is rejected and the k-space line is re-acquired (Fig. 16.4). There are multiple ways to implement navigator echo gating schemes and the gating can be done retrospectively or prospectively. The major applications of navigator echo gated PCMR are in time-resolved 3D imaging (so called 4D PCMR) [10], or in applications that require high spatial resolution such as coronary artery flow measurements [11].
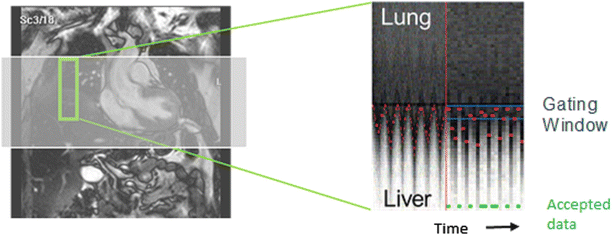
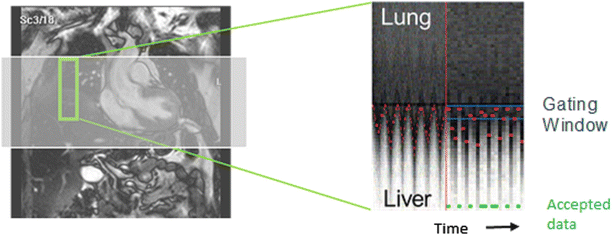
Fig. 16.4
Schematic illustrating navigator echo gating. Signal from a small region that contains the diaphragm is acquired every heart beat (or more often). The motion of the interface over time represents respiratory motion. The user may set the gating window to accept data from a certain portion of the cardiac cycle
Velocity Encoding Direction
The velocity encoding direction is independent of the slice orientation. Therefore, the velocity of blood (or tissue) can be encoded through the slice, or the velocity in either of the in-plane directions can be encoded. The direction of encoding can be set in the protocol which changes the direction of the bipolar velocity encoding gradient. Note that the velocity encoded in the image phase is the projection (or dot product) of the velocity vector in the direction of the encoding gradient. For example, if the PCMR image slice is off by 30° perpendicular to the velocity direction, then the displayed velocity will be the true velocity multiplied by cosine (30°), or 0.87, resulting in a velocity error of ~13 % from the true value.
Here it is important to understand the difference between velocity and flow. Velocity is the time-rate of change of position in a specific direction of fluid and has the units of length/time (i.e. cm/s). Flow is the rate of volume flux of fluid through a region per unit time and has the units of volume/time (i.e., milliliters/s). Flow requires velocity measurements to be integrated over a cross-sectional area. Therefore, flow can only be determined with through-plane velocity encoding, when estimating peak velocities, care must be taken to align the slice perpendicular to the velocity direction. Flow measurements are less susceptible to this issue as the increase in the vessel area compensates for the decrease in the value of the velocity vector due to misalignment.
Velocity Encoding Value (VENC)
The VENC value is a parameter set by the user and determines the time and magnitude of the applied bipolar velocity encoding gradients. The VENC essentially acts as a relation between the velocity values and the measured phase. If the measured phase exceeds +180°, or goes below −180°, it will be assigned a value within the 180° to +180° range since only 360° of phase can be measured. This condition is known as velocity aliasing or velocity wraparound, and an example from a patient is shown in Fig. 16.5. To avoid aliasing, a VENC value must be chosen so that aliasing does not occur. VENC values are displayed as the maximum velocity that can be measured without aliasing. This produces a difficulty in PCMR as the maximum velocity is not known a priori. When a good estimate of the maximum velocity cannot be made, a rapid scan can be done to check if aliasing is present. A VENC value that exceeds the maximum expected velocity but only by a small amount (~25 %) will maximize the ranges of phases used to measure velocity and hence increase the velocity-to-noise ratio. Additionally, it is important to realize that a smaller VENC value requires a larger gradient value, which will increase the echo time and may reduce the number of phases that can be acquired.
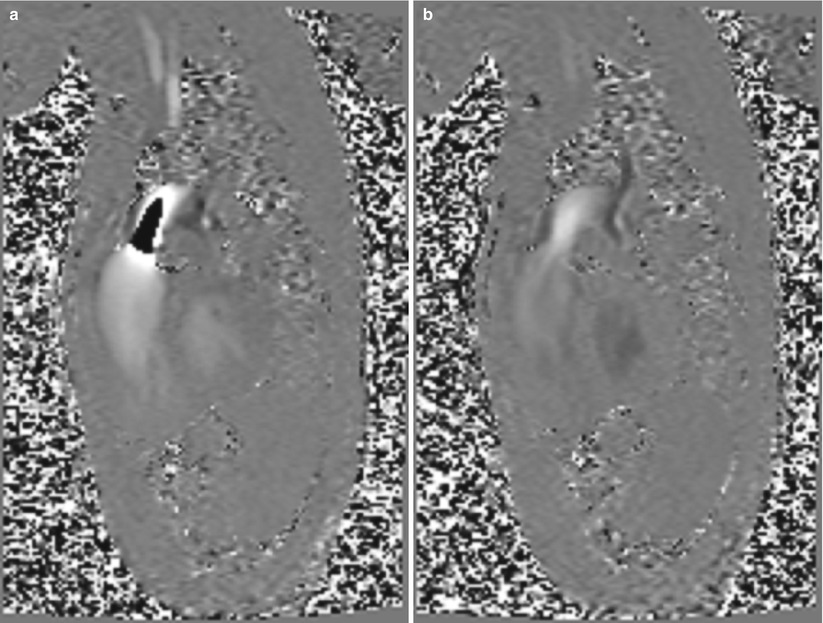
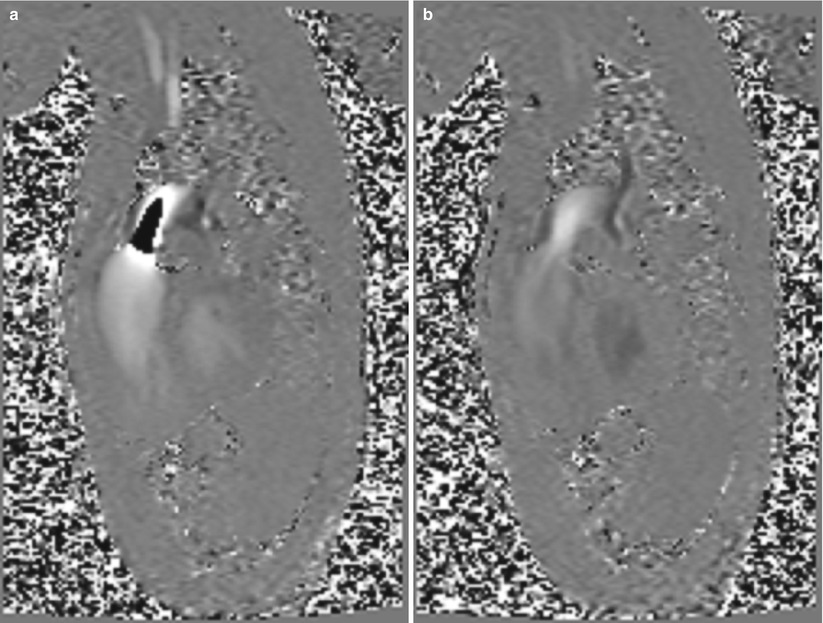
Fig. 16.5
Example of aliasing in the pulmonary artery. On the left the VENC value was set too low and the central jet of the flow appears dark, suggesting reverse flow (toward feet). The image on the right was taken with a higher VENC value and the aliasing disappears
Specialized Implementations of PCMR
The majority of PCMR examinations are conducted using a single slice, 2D, ECG gated, cine implementation with velocity encoded through the slice plane to determine vessel flow as described above. However, several recent developments have enabled new applications that expand on this basic implementation and these are described below.
Alternate k-Space Sampling Strategies
Most work in PCMR has been done using standard Cartesian k-space sampling. One of the major problems using PCMR to quantify blood flow is the lengthy acquisition times associated with the technique. Multiple methodologies have been used to reduce acquisition time. Techniques such as vastly under-sampled radial projection reconstruction (VIPR) can be combined with PCMR [12]. PC-VIPR can reduce acquisition time of PC velocity images by sparsely sampling the edges of k-space and more densely sampling the central regions of k-space with a constrained radial sampling strategy. Interleaved spiral acquisition has also been used to reduce temporal resolution of the PCMR velocity measurements. The spiral technique has been used in a single slice, single direction mode to improve temporal resolution. These rapidly acquired images can be used to monitor short duration physiologic changes in cardiac output [13]. A third method to improve the acquisitions time is to use parallel imaging techniques with or without constrained reconstruction.
4D PCMR
The PCMR described previously can be expanded to measure multiple time-resolved velocity directions over a 3D volume (4D PCMR). As previously mentioned, creation of a PCMR image requires two underlying images (flow-encoded and flow compensated). Obtaining multiple directions of velocity increases this time, but advanced encoding strategies can limit the time to a maximum to four times that of the standard gradient echo sequence [14]. Implementing the technique with a 3D acquisition volume allows the voxel size to be reduced over a standard 2D acquisition, and allows for an integrated volume acquisition. Therefore, 4D PCMR can be used to completely define the three-dimensional, time-resoled velocity vector field over a 3D volume in vessels. Having this complete, time-resolved velocity field enables advanced visualization such as plotting streamlines, pathlines, and particle traces. A variety of cardiovascular applications have been examined with 4D PCMR, including ventricular flow and carotid flow, but applications in aortic disease has been a primary focus of many investigators [15, 16]. The major drawback to widespread use of 4D PCMR is the time required for acquisition. Even with parallel imaging techniques, acquiring velocity within the aorta requires ≈10 min.
Tissue Velocity Mapping
Similar to what has been done in ultrasound with tissue Doppler imaging (TDI), investigators have used PCMR to map velocity of the left ventricular myocardium rather than the blood flow [17]. This requires a low VENC value and a fairly high spatial resolution acquisition. The disadvantage is that these low VENC values requires a high velocity encoding gradient to generate adequate phase for the low velocities seen in the myocardial tissue (typically <10 cm/s). The higher gradient increases echo time, often causing susceptibility artifacts in the LV lateral wall, and reduces overall SNR within the image [17–20]. Other issues include the lengthy acquisition time required to cover the entire LV, and issues with large phase shifts from the flowing blood with in the left ventricle. Several applications, including measurement of ventricular dyssynchrony have shown promising results [20].
Real-Time PCMR
Because of the need for subtraction of two images in PCMR, development of real-time PCMR has lagged behind other MRI-based real time applications, such as cine imaging of the LV function, or monitoring of needles or catheters in interventional procedures. Using EPI or spiral acquisition and shared encoding strategies have allowed investigators to use real time PCMR to monitor exercise or stress-induced changes in aortic hemodynamics, and diagnosis of congenital and acquired pathology [21]. Because of the complex acquisition strategies and the required post-acquisition subtraction, there is often a delay in displaying the ‘real-time’ PCMR data. Investigators have employed and external dedicated reconstruction hardware to improve the time between delay and acquisition [22, 23].
Pulse Wave Velocity
Pulse wave velocity (PWV) can be used as a measure of arterial stiffness through the Moens-Korteweg equation. The PWV is most often estimated by dividing the distance between two measurement sites by the propagation time of the pressure or flow wave between the two sites. Two slices perpendicular to the aorta are most commonly used for recording locations, but multiple locations using in-plane flow encoding can also be used. A time-marker on the flow waveform must be chosen in order to compare pulse wave arrival times at the sites. High temporal resolution in the PCMR images is critical as the wavespeed is 3–10 m/s in the aorta [24, 25].
Post-processing of PCMR Data
Background Phase Correction
Despite the subtraction of velocity-encoded and non-encoded images, a background phase offset may remain in static tissue, causing static tissue to appear to have a small velocity. The phase offset is caused by concomitant gradients in phase-and by residual eddy currents. The phase offsets can be dependent on position in the image, but generally are larger as the distance from the iso-center increases. Consideration of the background phase is important because a large background phase can affect the accuracy of flow measurements, especially when calculating quantities such as regurgitant fraction [26]. A variety of techniques have been employed to reduce the background phase or mitigate its effect on quantitative velocity measurements. From the acquisition side, it is important to keep the image slice and vessel near the iso-center of the magnet where effects of inhomogeneity and gradient eddy currents are minimal. From the post-processing side background phase effects can be removed with a background correction algorithm that can be automated, or use user-defined regions of interest place in static tissue.
Analysis of Velocity Images
By drawing a region of interest curve (ROI) around a vessel, statistics such as average velocity, peak velocity, and a velocity histogram can be determined in a region. One can multiply pixel area times the velocity value in each pixel to obtain flow for each frame. If the regions of interest (ROI’s) are drawn for each time frame acquired, one can determine flow as a function of time over the cardiac cycle. Care is usually taken to trace boundaries near the edges of the vessel to eliminate contamination from other vessels, and to eliminate errors in the integration due to inclusion of excess static tissue.
Flow versus time curves can be used to determine clinically relevant quantities such as aortic regurgitant volume, abnormal ventricular filling patterns, cardiac stroke volume, cardiac output, quantification of volumetric flow in left-to-right shunts [27]. Advanced calculations can be done on the velocity maps to create parametric images of wall shear stress, pressure gradient, vorticity, etc.
Clinical Application of PCMR
A multitude of CMR techniques can aid in delineating specific etiologie and pathophysiology. CMR provides gold standard assessment of ventricular mass, volumes, wall motion, and viability via various cine and contrast enhancement techniques. PCMR provides a complementary hemodynamic assessment of systolic and diastolic function, further aiding both diagnosis and prognostication in patients with various cardiac diseases.
Myocardial Disease
Clinical heart failure may arise from impaired myocardial contraction, relaxation, or both conditions. Phase contrast magnetic resonance (PCMR) is useful in assessing the degree of both systolic and diastolic dysfunction due to various myocardial diseases, as well as impaired ventricular filling. The contribution of various myocardial, valve or shunt lesions to pressure and/or volume overload and secondary myocardial dysfunction can also be evaluated by PCMR.
Cardiac Output
Cardiac output (CO), is the product of stroke volume (SV) and heart rate and is an important clinical parameter in the assessment of patients with heart disease. Reduced CO or cardiac index (CI defined as CO/BSA) is the hallmark of systolic or diastolic dysfunction from any etiology. Traditionally, the most accurate method of measuring CO has relied on invasive cardiac catheterization. CMR offers a non-invasive measurement of CO by both cine ventricular volumetry and PCMR, both with accuracy and precision as comparable to invasive measures. Both CMR methods have established reference values, although cine ventricular volumetry is limited in the case of regurgitant valve disease.
The method of PCMR CO or SV measurement relies on a through-plane velocity measurement and integration of flow volume in one or both of the great vessels as described above. For LVSV measurement, the ascending aorta should be localized in an oblique sagittal scout image to visualize patient specific architecture. The PCMR imaging plane should be set perpendicular to the direction of flow. The location of the imaging plane relative to the aortic annulus is a matter of debate, as some groups recommend a proximal position between the annulus and sinotubular junction, while others recommend the mid-ascending aorta at the level of the main pulmonary artery (PA) bifurcation. The proximal position may be more susceptible to inaccuracies related to valve motion artifacts and complex flow patterns, however, it avoids error related to diastolic coronary flow. Measurement in the mid-ascending aorta, although felt to be more reproducible, may be more affected by aortic compliance, which underestimates regurgitant volume, and reverse coronary diastolic flow, which may account for 0.5–6 % of aortic forward flow. The VENC should initially be set at 200 cm/s.
RVSV is measured in main PA. The main PA should be localized in an oblique axial or coronal scout image to visualize the curvature. The imaging plane should be set perpendicular to the direction of flow, between the pulmonic valve and main PA bifurcation. The VENC should initially be = 200 cm/s. Comparison of the SV of the left and right ventricle (RV) may be used to provide an internal control, or to calculate Left-to-right’s shunt ratio, if applicable.
Diastolic Dysfunction
Cardiac diastolic dysfunction is an abnormality of ventricular relaxation and occurs in a variety of heart failure etiologies. Diastolic heart failure is a predominant cause of heart failure symptoms and may precede or accompany systolic dysfunction. Diastolic dysfunction is also a marker of poor outcomes, as patients with diastolic heart failure and preserved systolic function have similar mortality to patients with isolated systolic dysfunction [28, 29]. Diastology is also useful in the clinical management of patients, notably in the estimation of LV filling pressures and guidance of medical therapies.
Clinical diastolic function evaluation is most often performed with Doppler transthoracic echocardiography (TTE). Conventional parameters determined include early and late filling peak velocities of the transmitral flow (E and A waves), E-wave deceleration time (DT), and annular myocardial early longitudinal peak velocity (E′) and late longitudinal peak velocity (A′). Pulmonary vein peak systolic (S) and diastolic (D) velocities are a useful adjunct, specifically in the form of the S/D ratio.
CMR can offer a comprehensive evaluation of diastolic function comparable to Doppler TTE with the use of PCMR. PCMR derived diastolic hemodynamics will provide useful information in patients planned to undergo CMR or those with poor TTE windows. Additionally, PCMR may overcome certain limitations of Doppler TTE, including limited field of view, beam angle dependency, and incomplete sampling of eccentric flows.
Transmitral Flow Profiles
Cine CMR imaging in multiple long axis views should be used to visualize the mitral leaflets for planning a PCMR slice location to evaluate transmittal velocity. The PCMR slice should be prescribed at the leaflet tips during diastasis, parallel to the plane of the mitral annulus. Through-plane images should be acquired with retrospective EKG gating. An initial VENC setting around ~200 cm/s is reasonable. However, the correct VENC may vary, between 150 and >300 cm/s, depending on the patient’s hemodynamics. After image acquisition, the ROI is traced around the inner contour of the mitral valve (MV) orifice in cross-section on magnitude images, and then transferred to phase images.
Transmitral peak E and A velocity waves are plotted on a flow versus time graph. The DT is calculated by subtracting the time of intersect of the peak E wave with the baseline from the time of intersect of the E wave downslope with the baseline. PCMR derived peak E and A velocity waves correlate well with Doppler TTE techniques, although there is a tendency towards underestimation of magnitude. Assessment of transmitral profiles and E/A ratio, however, have excellent agreement with Doppler TTE for identifying the presence and stage of diastolic dysfunction [30, 31].
Pulmonary Vein Flow Waveform
The right superior pulmonary vein is often the easiest to acquire on cine CMR or scout images, however, any or all visualized pulmonary vein may be imaged. The slice plane should be perpendicular to flow inside the vein, approximately 1 cm from the ostium. Through-plane PCMR images should be acquired with retrospective EKG gating and a VENC of 100 cm/s. After image acquisition, the ROI is traced around the inner lumen of the pulmonary vein on magnitude images, and then transferred to phase images. Pulmonary vein S and D average velocity waveforms are plotted on a velocity versus time graph to the time-dependent characteristics of the flow. PCMR derived pulmonary vein S/D ratio correlates well with Doppler TTE [32].
Mitral Annular Tissue Velocity
The basal lateral and/or basal septal segments of the mitral annulus can be visualized on long axis cine CMR imaging. A PCMR slice to measure mitral annular velocity should be placed at the lateral and/or septal MV annulus, with care to ensure it the slice does not include mid-left ventricular or left atrial myocardium. Through-plane PCMR images should be acquired with retrospective EKG gating and a VENC of 50 cm/s. After image acquisition, the ROI in the annulus is traced on magnitude images and transferred to phase images.
Mitral annular tissue average velocity waveforms are plotted on a velocity versus time graph to determine time-dependent velocity behavior of the tissue. PCMR derived mitral annular tissue velocities have been found to correlate well with tissue Doppler TTE [30, 33]. The E/E′ ratio has been found to correlate well between PCMR and Doppler TTE, as well. Importantly, PCMR derived E/E′ correlates strongly with invasive pulmonary capillary wedge pressure by cardiac catheterization with patients with E/E′ <8 have PCWP <15 mmHg and those with E/E′ >15 having PCWP >15 mmHg [20, 34].
Restrictive Physiology
Restrictive physiology may be a result of progressive diastolic dysfunction or restrictive cardiomyopathy. Restrictive cardiomyopathy often presents with signs of right heart failure including peripheral edema and ascites. Imaging findings include normal or reduced ventricular volumes and normal or near normal ventricular systolic function, often with significantly dilated atria. In this clinical setting, it is important to distinguish restrictive and constrictive physiology. Severe abnormalities of myocardial relaxation and filling, with E/A >2 and mitral annular tissue velocity <4 cm/s, is categorized as restrictive physiology. Moreover, CMR morphologic assessment and tissue characterization provides further analysis of specific causes of restrictive cardiomyopathy such as amyloidosis, sarcoidosis, hemochromatosis, Fabry’s disease, endomyocardial fibrosis, and carcinoid heart disease [35].
Pitfalls
Diastolic transmitral hemodynamic profiles and mitral annular tissue velocities may be inaccurate due to large through-plane motion of the annulus over the cardiac cycle. Specifically, transmitral diastolic velocities and flow may be underestimated. Visual confirmation and manual redrawing of the ROI at each cardiac phase to ensure the appropriate location over each cardiac cycle during post-processing may minimize error. Annular tracking with long-axis cine CMR navigators may provide motion compensation, although this technique is not widely available. The underestimation of peak E and A wave velocity magnitude with PCMR may be related to increased sensitivity of maximal velocity curves to noise or the temporal resolution of PCMR.
Pericardial Disease
CMR provides an accurate assessment of pericardial morphology including thickness, pericardial effusion, acute inflammation and fibrosis. CMR can also provide complementary functional and hemodynamic information on cine PCMR sequences.
Pericardial Constriction
Pericardial constriction occurs when normal ventricular diastolic filling is impeded by a non-compliant pericardium. In the clinical setting, it is critical to distinguish restrictive and constrictive physiology. Although pericardial thickening and even calcification may be present. This finding is not diagnostic of constriction, as constriction may exist without either finding [36, 37].
The hemodynamic hallmark of constriction is dissociation between intracardiac and intrathoracic pressures evidenced by ventricular interdependence and respiratory variations in atrioventricular valve inflow velocities, typically evaluated by Doppler TTE. CMR can provide an assessment similar to that of TTE, including volumetric and functional evaluation, identification of the interventricular septal bounce suggestive of ventricular interdependence and, inferior vena cava (IVC) plethora. Thorough hemodynamic evaluation is essential, as the interventricular septal bounce and IVC plethora may be seen in a variety of other conditions.
Pitfalls
Mitral and tricuspid annular motion with the cardiac cycle and respiration may displace the desired imaging plane on the horizontal long axis images, potentially introducing error into the flow measurements. This can be minimized by visual confirmation of enface inflow orientation with each frame during acquisition and redrawing of the ROI to ensure the appropriate location during post-processing.
Although the use of free-breathing acquisition improves temporal resolution, this may come at the expense of reduced signal-to-noise ratio. Furthermore, maximal velocity curves may have increased sensitivity to noise as they represent single time point in the cardiac cycle, compared to flow rates, which are estimated from the averaged velocity throughout the cycle. However, given the measurement of respiratory cycle-based velocity variation occurring over hundreds of milliseconds, this is unlikely to have significant impact.
Valve Disease
Left sided valve disease imposes a progressive hemodynamic burden on the heart in the form of pressure overload, volume overload, or both. Established criteria guide physicians in treating patients with left sided valve disease by incorporation of symptoms and cardiac structural changes, in addition to lesion severity as assessed by various imaging techniques. Quantitative assessment of lesion severity is preferred, and may facilitate intervention at an earlier stage prior to potentially irreversible structural changes and limiting symptoms. PCMR provides an accurate non-invasive estimate of hemodynamic profiles across any valve or vascular structure, and allows for precise quantitative results that may be complementary to the anatomic findings of both TTE and cine CMR. Transvalvular velocities, pressure gradients, flow rates, and flow volumes can be directly measured or calculated.
Aortic Stenosis
Aortic stenosis (AS) due to calcific degeneration is the most common valve disease of the elderly. However, congenital and rheumatic aortic valve (AV) disease may present as significant stenosis in children and young adults. Regardless of etiology, progressive narrowing of the AV orifice results in pressure overload of the LV with associated concentric remodeling and elevation of LV filling pressures. Surgical treatment decision is based on lesion severity and patient symptoms. AS is considered severe when the area of the valve orifice is less than 1 cm2, the peak velocity is greater than 4 m/s, or the mean transvalvular gradient is more than 40 mmHg. PCMR imaging is useful in determining these structural and hemodynamic findings. Generally, the VENC should be based on the initially estimated severity and can be subsequently adjusted based on the presence of aliasing in the images. Cine CMR also provides complimentary structural assessment of valvular morphology, such as cusp number and fusion, and ventricular remodeling related to chronic pressure overload. Dynamic characterization of the valve orifice area, including morphology (i.e. bicuspid versus tricuspid) and direct measurement of the valve area is possible with PCMR. This method relies on visualization of a distinct boundary identifying high velocity pixels of the jet. The imaging plane should be parallel to the AV annulus and use a short TE, high temporal resolution cine PCMR sequence.
< div class='tao-gold-member'>
Only gold members can continue reading. Log In or Register a > to continue
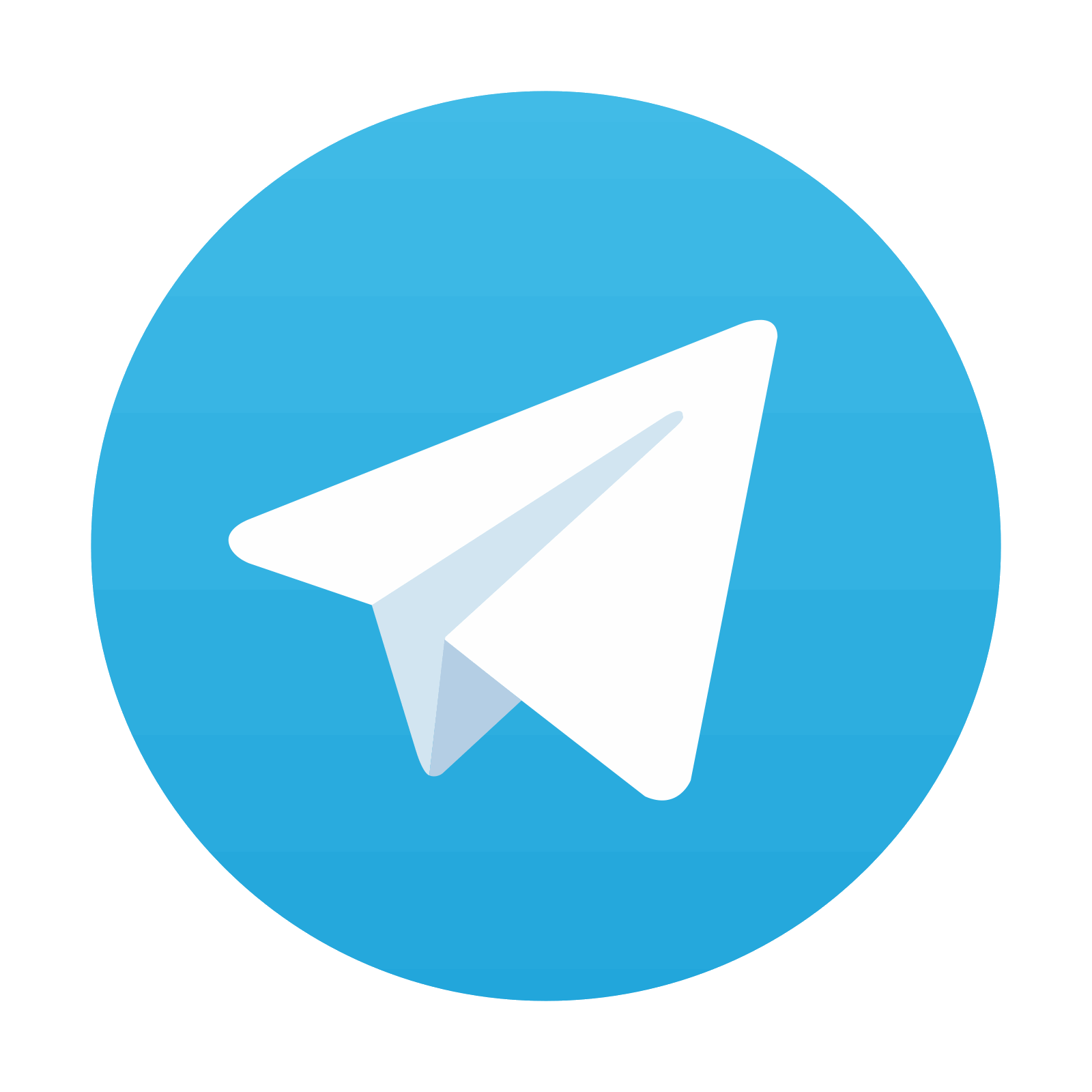
Stay updated, free articles. Join our Telegram channel
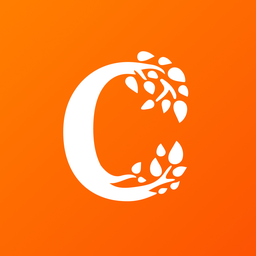
Full access? Get Clinical Tree
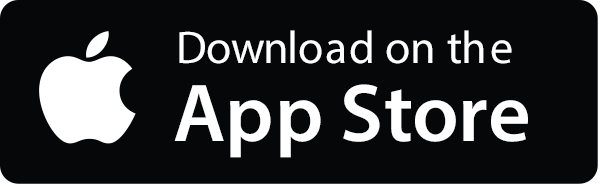
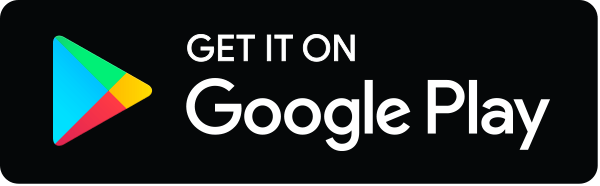