Fig. 5.1
Reducing TR. Several different ways to reduce the TR in a standard Fast Low-Angle SHot (FLASH) sequence, shown in (a): Shorter gradient durations with higher gradient strengths can be used (b), the duration of the RF pulses can be shortened (c), or the acquisition bandwidth can be increased (sampling duration decreased) (d)
It is important to note that both the echo time, TE, and the repetition time can be shortened using these methods, and both can affect the image contrast. However, shortening the echo time without reducing the repetition time will not shorten the overall duration of the sequence, and thus reducing the TR is the focus of this section.
In order to shorten the TR while maintaining the gradient moments, higher gradient amplitudes and slew rates must be used (as in Fig. 5.1b). For instance, in order to halve the amount of time required for gradient encoding, the gradient amplitude has to be doubled, and the slew rate has to be quadrupled. While challenging, such gradient performance is possible given recent engineering advances, but the potential for peripheral nerve stimulation caused by rapidly changing fields limits the gradients that may be applied in a clinical examination. Current clinical sequences operate close to this limit, making further improvements in imaging speed through increased gradient amplitudes unlikely.
Another way of reducing the TR is to optimize the RF pulses used for slice excitation (the influence of a shorter RF pulse on TR is shown in Fig. 5.1c). A perfectly rectangular slice can only be selected using an infinitely long sinc pulse, and thus truncated sinc pulses with a considerably shortened duration but fewer side lobes are applied for slice selection. However, the truncation of the excitation pulse leads to a softening of the rectangular shape of the excited slice and a coarser slice definition. Instead, it is possible to use a high excitation bandwidth and a large slice selection gradient and compress the RF pulse. Such a compressed RF pulse has the same number of side lobes as an uncompressed RF pulse and leaves the profile of the selected slice unaffected, but the compression is limited by the maximum slice select gradient strength. The amplitude of the RF pulse must also be increased in order to shorten the RF duration while maintaining the flip angle. As larger amplitudes result in a higher deposition of energy which can potentially cause tissue heating, care has to be taken to limit the RF transmitter output. Another option is to use an asymmetric RF pulse, in which only one side of the RF pulse is truncated. In this case, a higher number of side lobes can be employed (on one side of the pulse) which can lead to an improved slice profile over a symmetric RF pulse with the same duration.
The third set of related parameters which influence the sequence timing includes the dwell time and receiver bandwidth (rBW) used for data collection. The dwell time describes the interval with which data points are sampled during the readout, and the rBW is the inverse of the dwell time. In general, the time needed to collect each line of k-space can be reduced by decreasing the time between sampled data points (in other words, increasing the rBW, as shown in Fig. 5.1d). If the total time needed for data sampling is reduced, the whole pulse sequence can be condensed, allowing the use of shorter TE and TR. However, an increase in rBW leads to a reduction in the signal-to-noise ratio (SNR), and the amount by which the scan can be accelerated by increasing the rBW is limited by the maximum read gradient strength. Another option is to employ an asymmetric readout, in which different numbers of readout points are collected before and after the echo, which can help shorten the readout portion of the pulse sequence.
If further reduction of the TR using approaches based on those described above is limited, for instance by nerve stimulation or specific absorption rate (SAR) related tissue heating, another option to shorten the scan time is to reduce the spatial resolution. The total scan time is directly related to the number of k-space lines collected, which is in turn directly related to the spatial resolution and the FoV. As the FoV is determined by the size of the patient, the only free parameter is the spatial resolution. Thus, if a lower spatial resolution can be tolerated, some k-space lines can be eliminated. While this trade-off between spatial and temporal resolution is inherent to all MRI acquisitions, it is of particular importance in cardiovascular MRI where moving anatomy limits the amount of time available for data collection. If no spatial resolution can be sacrificed, a longer scan with a lower temporal resolution must be used, and any motion that occurs during data collection can lead to artifacts.
Cardiac and Respiratory Synchronization
Although reducing the TR is an effective way to reduce the acquisition time, an approach often used to avoid artifacts when scanning in the presence of cardiac motion is to synchronize the acquisition to the patient’s electrocardiogram (ECG). The ECG signal can be obtained using ECG electrodes and leads attached to the patient’s chest. The QRS complex is detected and employed to trigger the start of the imaging sequence. The time between the QRS detection and the start of the sequence is called the trigger delay and can be adjusted such that data are collected only when the heart is in the desired cardiac phase. By distributing the acquisition over multiple heartbeats and changing the phase encoding each time data are collected, a motion-free image of the selected cardiac phase can be reconstructed.
Using conventional cardiac synchronization as described above, only one line of k-space is acquired per heartbeat. In these cases, the repetition time, TR, is equal to the R-R interval of the patient. The acquisition of a complete dataset depicting one cardiac phase using conventional spin echo (SE) or gradient echo (GRE) sequences can take up to several minutes. For an image with a spatial resolution of 2 mm2 and a FoV of 256 mm2 acquired in a patient with a heart rate of 80 beats per minute (TR equals one heartbeat, or 0.75 s), data collection would take 1.6 min (128 phase encoding lines with a TR of 0.75 s). Because respiratory motion will change the position of the heart and large parts of the thorax, the reconstructed images would be strongly affected by motion artifacts, and respiratory compensation methods would have to be applied.
The simplest and therefore most common method for avoiding respiratory motion artifacts is to ask the patient to perform a breath-hold during data acquisition. In cases where patients have problems holding their breath long enough for the scan to be performed, the sequence can be triggered by a respiratory bellows wrapped around the patient’s chest. The breathing motion of the chest leads to expansion and contraction of the bellows, which can be detected for respiratory gating or compensation. As in cardiac gating, respiratory gating involves using the respiratory signal to directly trigger the start of the sequence. Data are then only acquired when the heart and lungs are in the proper cardiac and respiratory phases. In respiratory compensation, the respiratory signal is recorded in order to retrospectively select the k-space lines that are sampled within the selected portion of the respiratory cycle. Both methods require the patient’s respiration to remain regular throughout the scan, and can lead to long scan times.
From Single-Echo to Multi-echo Acquisitions
In single-echo SE or GRE sequences, one RF excitation pulse is combined with a series of other RF pulses and gradients to create a single echo. In these sequences, one line of k-space is acquired in each TR, followed by a delay time to allow the longitudinal magnetization to recover. The delay time depends on the tissue-dependent longitudinal relaxation time, T1, and can be on the order of seconds for SE sequences, leading to extremely long TR unsuitable for cardiac imaging. Additionally, if only one k-space line is encoded for each heartbeat and breath-holding is used to reduce respiratory motion, the number of k-space lines and therefore image resolution would be severely restricted by the breath-holding time.
Accelerating Single-Echo GRE Sequences
In GRE sequences, gradients are used instead of RF pulses to refocus the magnetization to form an echo. The generation of the gradient echo can be performed quite rapidly (on the order of a few milliseconds), but the TR is determined by the time needed for the recovery of the longitudinal magnetization as well as for the dephasing of the residual transversal magnetization. A typical pulse diagram of a conventional GRE sequence is shown in Fig. 5.2a. Instead of waiting for T2 relaxation to dephase the transversal magnetization, there are two widely used techniques to significantly reduce the waiting time needed before the next excitation can be performed:
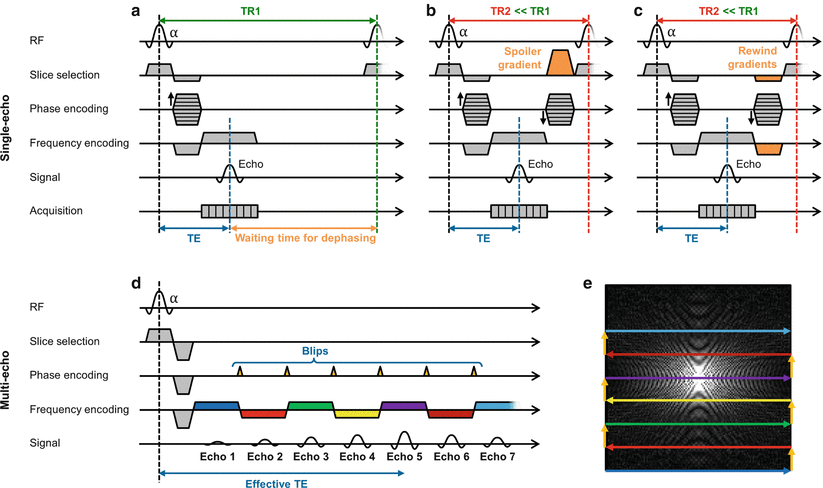
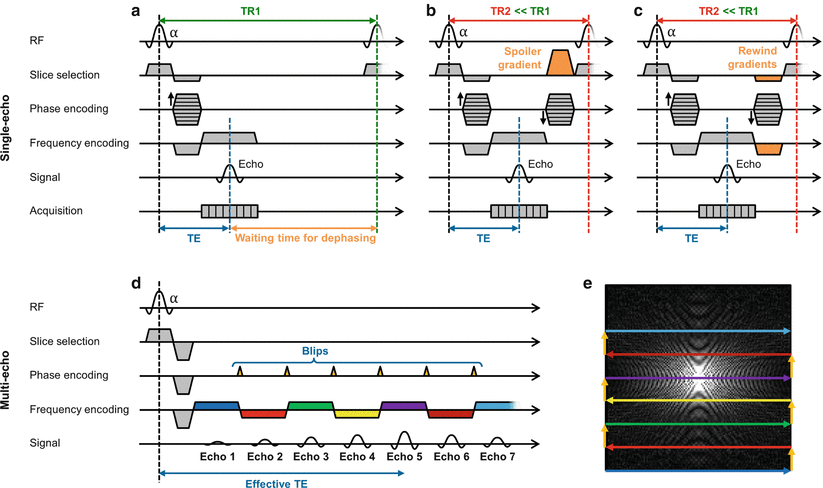
Fig. 5.2
Accelerating GRadient Echo (GRE) sequences. Sequence diagram of a conventional gradient echo sequence (a). The repetition time, TR, in a single-echo GRE sequence can be reduced by using spoiler gradients (b) or rewinding gradients (c). Multiple echoes can be created using an Echo-Planar Imaging (EPI) sequence (d): diagram, and (e): corresponding k-space pattern. After the excitation pulse, the alternation of repeatedly reversed readout gradients and small phase encoding gradients (blips) generates a series of echoes. The echo with the smallest gradient moment (echo 5 in the diagram) defines the effective echo time TE
Spoiled gradient echo sequences (General Electric: SPoiled GRadient echo, SPGR | Philips: T1 Fast Field Echo, T1 FFE | Siemens: Fast Low-Angle SHot, FLASH) apply spoiler gradients to ensure that the transversal magnetization from one excitation is completely dephased before the next excitation [1–3]. Subsequently, successive excitations can be performed without any additional recovery time. Relatively small flip angles are typically used to ensure that enough longitudinal magnetization will be present for excitation despite the short TR used. Because the longitudinal magnetization is greatly reduced when using FLASH sequences, there is a corresponding loss in SNR.
Instead of dephasing the remaining transverse magnetization, rewound or balanced gradient echo sequences (introduced as balanced Steady-State Free Precession, bSSFP | General Electric: Fast Imaging Employing STeady-state Acquisition, FIESTA | Philips: Balanced Fast Field Echo, b-FFE | Siemens: TrueFISP) apply rephasing gradients of opposite signs to rewind any residual transverse magnetization left after data collection [4]. After every excitation, these coherent transverse components add to the “freshly” excited transverse magnetization. This leads to a steady-state with a higher signal than FLASH sequences, but also a more complicated contrast, with a combination of T1 and T2 weighting. Additionally, the avoidance of lengthy dephasing times enables a larger reduction of the TR compared to FLASH. The combination of high SNR and excellent contrast between blood and myocardium make bSSFP sequences among the most widely used in cardiovascular MRI.
The use of spoiling or balanced gradients are two of the most effective ways of accelerating gradient echo sequences. In the case of an image with a spatial resolution of 2 mm2 and a FoV of 256 mm2, the use of a fast GRE sequence would typically result in a reduced TR of about 3 ms, and data collection could be performed in about 0.4 s (128 phase encoding lines with a TR of 3 ms). With such acquisition times shorter than the cardiac cycle period, these sequences can be used without additional acceleration during diastole to generate images with minimal motion artifacts.
Multi-echo Sequences
An efficient way to further accelerate the acquisition process is to generate multiple echoes after a single excitation, which drastically reduces the number of TR intervals needed to acquire an image and enables the acquisition of several k-space lines in rapid succession. These multi-echo extensions exist for spin echo as well as gradient echo based sequences and are known as turbo or fast pulse sequences.
Turbo Spin Echo/Fast Spin Echo
In the standard SE sequence, one RF excitation pulse is combined with an RF refocusing pulse to generate one spin echo, which is encoded to form one line of k-space. The fast/turbo spin echo sequence (introduced as: Rapid Acquisition with Relaxation Enhancement, RARE | General Electric: Fast Spin Echo, FSE | Philips, Siemens: Turbo Spin Echo, TSE) is a modification of the standard SE sequence which employs additional RF refocusing pulses to generate several spin echoes, called an echo train [5, 6]. The pulse diagram of a typical TSE sequence is illustrated in Fig. 5.3. The number of echoes within the echo train is often denoted as the turbo factor, TF (Philips, Siemens), or echo train length, ETL (General Electric), and the time between echoes as the echo spacing, ES. By changing the magnitude of the phase encoding gradient applied to each of these echoes, several different k-space lines can be encoded and collected. Because a total of TF k-space lines can be collected after one RF pulse, the scan time can be shortened by a factor of TF. This acceleration is often used to reduce the number of heartbeats needed for data collection, making data acquisition during a single breath-hold possible. If TF = 16, and a total of 128 k-space lines are required, only eight heartbeats are needed to collect all of the data for the image. This would reduce the acquisition time from 1.6 min to 6 s (from 128 to 8 heartbeats at 80 beats per minute). However, the total amount of time required to collect all TF lines of k-space is TF*ES. For instance, if a turbo factor of TF = 16 is used, and the ES is 3 ms, the total “acquisition window” is 48 ms. This “acquisition window” must be kept short enough to avoid image artifacts due to cardiac motion, and to minimize relaxation effects.
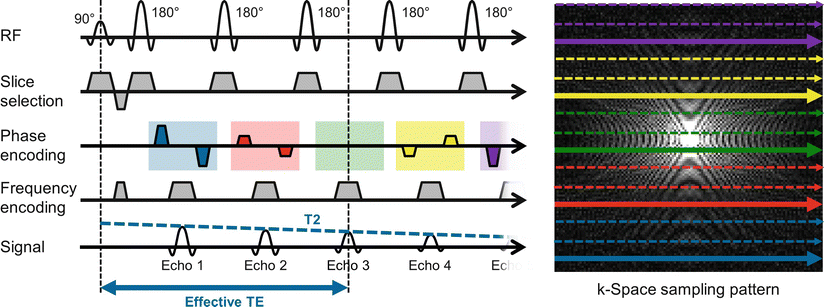
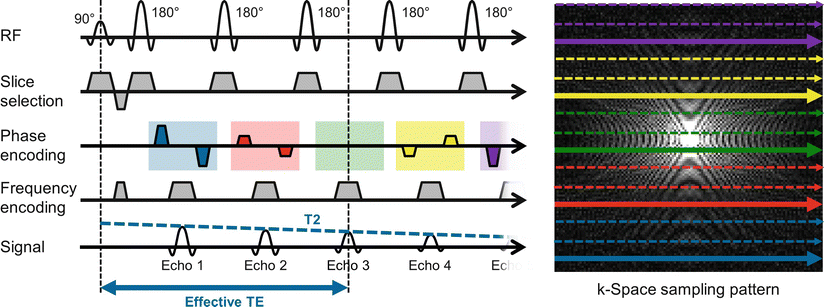
Fig. 5.3
Spin Echo (SE) to Turbo Spin Echo (TSE). Sequence diagram of a TSE sequence (left) with an echo train length (ETL) of 5, and the corresponding k-space sampling pattern (right) where the lines collected in one TR are shown in bold. After the 90° excitation pulse, the repeated application of 180° refocusing pulses generates a series of echoes. The echo with the smallest gradient moment (echo 3 in the diagram) defines the effective echo time TE. The TSE sequence is repeated until the k-space is filled; three shots are needed in this case. Note the interleaving of the acquired k-space lines to avoid discontinuities in k-space due to T2 relaxation
When a spin echo train is used, the echo time TE, and thus the T2 weighting, changes with each k-space line collected. For TSE sequences, an effective echo time is defined as the TE of the k-space line that is acquired closest to the center of k-space, which carries the majority of the image contrast. Because different k-space lines have slightly different contrasts, blurring can occur in the phase encoding direction due to T2 decay if the echo train is long. Similarly, if significant relaxation occurs during the collection of the echoes, the resulting images can have a low SNR. Another important consideration is the potential for discontinuities in k-space if significant differences in T2 relaxation exist between the end of one echo train and the start of the next. To avoid such discontinuities, which can lead to artifacts, k-space is generally filled in an interleaved fashion to avoid large T2 contrast jumps which could occur if a long echo train is used and the k-space filled sequentially.
Besides the extreme reduction in scan time, an additional advantage of TSE sequences is a signal increase in some tissues compared to conventional SE sequences. The reason lies in the interaction between hydrogen nuclei in molecules with long carbon chains known as J-coupling, usually reducing T2 relaxation times. The rapidly repeated 180° pulses of TSE sequence break up this interaction, leading to longer effective T2 times and therefore an increase in signal.
Single-Shot TSE/FSE
The highest acceleration possible for a TSE sequence can be achieved when the TF is equal to the number of k-space lines required for the entire image. This extreme case is known as single-shot TSE. For multi-slice acquisitions, the single-shot TSE sequence is applied sequentially to acquire one complete slice in a single heartbeat, before moving on to the next one. Due to the extremely high TF, significant T2 decay occurs during the acquisition of one slice, and unless combined with additional steps to shorten the echo train, such as partial Fourier acquisition, its clinical use is limited to imaging of primarily fluid structures with extremely long T2 relaxation times.
Multi-echo GRE: Echo-Planar Imaging (EPI)
Due to the possibility to dramatically shorten the repetition times in GRE based sequences with gradient spoiling and balancing in FLASH and bSSFP, these single-echo sequences are used in most cardiac imaging applications. However, as in single-shot TSE, it is possible to obtain multiple echoes after a single RF excitation pulse in GRE based sequences using Echo-Planar Imaging (EPI, [7]) which is illustrated in Fig. 5.2d–e. After the excitation pulse, the alternation of repeatedly reversed readout gradients and small phase encoding gradients (blips) generates a series of echoes. The timing of the echo with the smallest gradient moment defines an effective TE determining the contrast of the image. Although EPI sequences enable the acquisition of an entire image in only a fraction of a second, the acquisition scheme is prone to a variety of artifacts such as image distortions and signal dropouts that limits the use of single-shot EPI in cardiac MRI. However, segmented EPI, sometimes called hybrid gradient echo – echo planar imaging (GRE-EPI), in which only a relatively few echoes are acquired after each RF pulse, has found utility in CMR for first-pass perfusion and velocity quantification. A more detailed description of EPI sequences can be found in [8].
Segmented Acquisitions
As described above, a combination of cardiac and respiratory synchronization can be used to obtain motion-free images of a desired cardiac phase. The main disadvantage that TR depends on the relatively long heart rate and T1 relaxation can be overcome using the fast imaging sequences described in the previous section. Instead of using conventional GRE or SE sequences, which can produce only one echo per heartbeat, more rapid sequences such as TSE, FLASH or bSSFP can be used to generate multiple echoes in every heartbeat. By using the shortest TR possible, a group of k-space lines (typically referred to as one shot) can be acquired while the heart is in the desired cardiac phase. This series of events is repeated over successive heartbeats to acquire different groups of k-space lines until an entire k-space corresponding to the desired cardiac phase is filled. As these methods divide the acquisition of one image into multiple segments, they are often referred to as segmented measurements. Most importantly, segmented measurements are usually short enough that they can be performed during a breath-hold, greatly reducing motion artifacts.
Cine Imaging
Instead of acquiring only one cardiac phase over successive heartbeats, ECG gated segmented measurements can further be extended by acquiring partial k-spaces of multiple phases of the cardiac cycle (the so-called cine frames) during every heartbeat. This principle is illustrated in Fig. 5.4. As in segmented imaging, the k-space lines acquired in every group and every cardiac phase (indicated by different colors) are changed for consecutive heartbeats until a full k-space is available for each cine frame. The images of all cardiac phases can be viewed as a movie sequence or cine, allowing functional assessment of the heart and its wall motion, and a visual, qualitative assessment of blood flow.
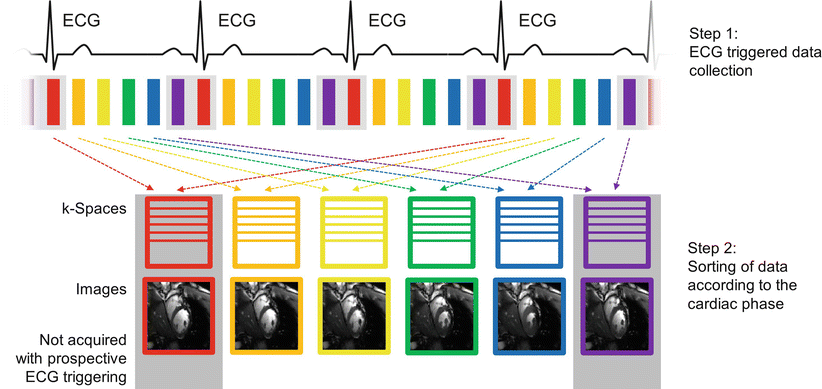
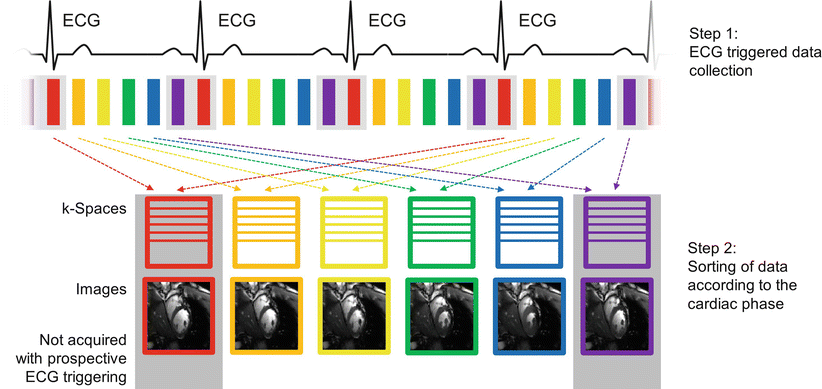
Fig. 5.4
Cine imaging. Prospective triggering: After an ECG triggered acquisition, the data are sorted into different k-spaces according to the acquisition order (indicated by different colors). Different parts of the k-space are filled in subsequent heartbeats. Because the prospectively triggered acquisition requires a small time window at the end of diastole to accomodate arrhythmic heartbeats (indicated by the gray box around the red and violet cardiac phases), these phases of the cardiac cycle are lost for image acquisition. Retrospective gating: Data are continuously acquired over multiple heartbeats. The ECG signal is recorded during the entire acquisition, and the data can retrospectively be sorted according to their cardiac phase. This enables imaging all cardiac phases including end-diastolic cine frames
The number of cine frames depends on the number of k-space lines collected per cardiac phase and the subject’s heart rate, which determines the total acquisition window. For example, if the subject has a heart rate of 80 beats per minute, the acquisition window is 750 ms. If 16 k-space lines with TR = 3 ms are collected per cardiac phase, each frame requires 48 ms (16*3 ms) of data collection time per heartbeat and approximately 15 cine frames (750 ms/48 ms) can be defined. To fill up a complete k-space with 128 lines, a total of eight heartbeats would be required. Acquiring a larger number of k-space lines for each cardiac phase leads to both a reduced temporal resolution, as a ‑longer time is needed to collect all of the lines, and a reduced number of cine frames, potentially leading to artifacts due to cardiac motion. Taking the previous example, if 32 k-space lines are collected for each cardiac phase in each heartbeat, the data acquisition for each cardiac phase would be 96 ms (32*3 ms), and only 7 cardiac phases could be collected. However, the overall acquisition time would be shorter, as fewer heartbeats (only four) are required to fill up the respective k-spaces. Because the total acquisition time is typically limited by the length of time the patient can hold his or her breath, an optimal balance between the number of cardiac phases to be acquired and the temporal resolution should be found by the operator.
Because cine imaging relies on very short repetition times, TR, it can only be used in conjunction with gradient echo based sequences such as FLASH and bSSFP. The particular choice depends on the field strength and the specific application.
Prospectively Triggered Cine Imaging
In prospectively triggered cine imaging, the acquisition is started at the R-wave of the ECG and no trigger delay is used in order to maximize the acquisition window. Typically, the last 10 % of the cardiac cycle is not included in the acquisition window and thus not used for sampling to allow for variations in the R-R interval. This period is known as the arrhythmia rejection (AR) window. For a heart rate of 80 beats per minute (an R-R interval of 0.75 s), a TR of 3 ms and a shot consisting of 16 k-space lines per cine frame, a total of 14 cine frames could be acquired in every heartbeat (90 % of 0.75 s time window divided by an acquisition time of 16*3 ms for each shot). The AR window is indicated by the gray boxes during end-systole in Fig. 5.4. In prospective gating, this part of the cardiac cycle is lost for image acquisition, and diastolic features of the cardiac cycle such as atrial contraction can be missed.
Retrospectively Gated Cine Imaging
A major disadvantage of prospectively triggered cine imaging is that the AR window between the end of the acquisition and the next trigger pulse is lost for image acquisition. One way to overcome this problem is to use retrospective gating [9], where data are continuously acquired over multiple heartbeats. By recording the ECG signal, each line of k-space can retrospectively be sorted into separate k-spaces corresponding to predefined cardiac phases. This results in a set of cine frames covering the entire cardiac cycle without the AR gap present in prospectively triggered acquisitions. This is again illustrated by Fig. 5.4, where the two end-diastolic cine frames indicated by the gray boxes would now be included by the acquisition. The main challenge of this gating technique is the retrospective binning of the acquired data. For arrhythmic heartbeats, systole usually remains relatively constant while the duration of diastole varies. Linearly stretching or contracting the data is therefore physiologically not realistic and has to be replaced by computationally more complex models. Alternatively, data acquired during significantly abnormal heartbeats can be re-acquired, but this can result in long breath-hold times, and increases the potential for respiratory motion artifacts. Nevertheless, retrospectively gated cine imaging is indispensable for imaging mitral tricuspid valve function or atrial contraction. For the same heart rate, shot size, and TR used in the prospective triggering example, a total of 15 cine frames per heartbeat could be acquired using retrospective gating, adding one end-diastolic frame to the cine movie sequence.
Reducing the Number of k-Space Lines
In addition to optimizing sequences and using segmented imaging to reduce the acquisition time, there are a few easy ways to speed up the acquisition by reducing the number of k-space lines collected. In this section, techniques which rely on the acquisition of fewer k-space lines will be explained along with their advantages and disadvantages.
Zero-Filling
The simplest way to speed up the acquisition is to acquire fewer phase encoding lines in the k-space periphery. Because the extent of k-space determines the image resolution, such an image would have a reduced spatial resolution in the phase encoding direction. However, the acquisition time is reduced by a factor equal to the proportion of phase encoding lines which were not collected. For example, if 16 phase encoding lines at both edges of a fully sampled k-space of 128 lines are skipped, the acquisition would be accelerated by 25 %.
Instead of directly using the smaller k-space and the lower resolution image, the missing parts of k-space are often filled with zeros before Fourier reconstruction in order to maintain the desired matrix size. This operation is equivalent to interpolating the original image with a small imaging matrix to fill a larger image matrix. As no information is generated in this process, the resulting image will have a larger matrix size but the actual image resolution will not be increased. An example where only one fourth of the central k-space is used for reconstructing the full FoV image with zero-filling is shown in Fig. 5.5b. A deterioration of the resolution in the phase encoding direction is clearly visible.
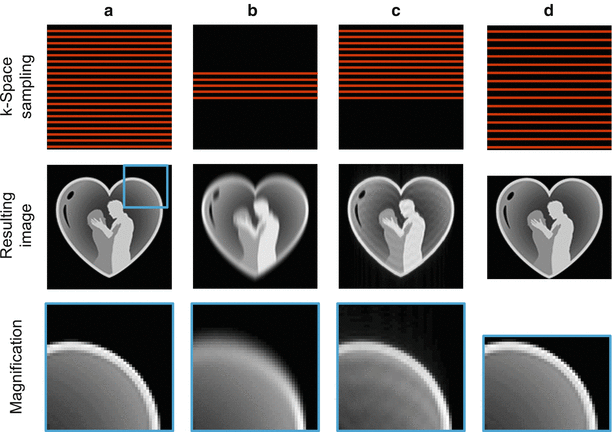
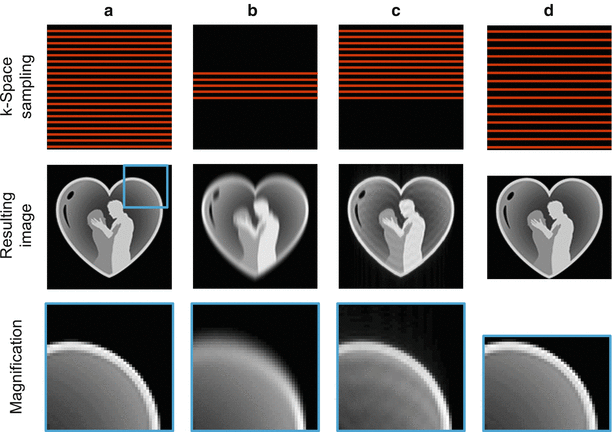
Fig. 5.5
Reducing the number of k-space lines. A fully-sampled k-space (top) and image (bottom) are shown in (a). Simply omitting outer parts of k-space during data collection, and replacing these pixels by zeroes to maintain the digital resolution, leads to an irreversible loss in spatial resolution (b). Instead, only slightly more than half the k-space is sampled in partial Fourier imaging, resulting in undersampling artifacts which can be greatly reduced by exploiting k-space symmetry (c). By acquiring fewer k-space lines spaced further apart (d), the FoV can be made smaller in the phase encoding direction to better fit the object and save scan time (rectangular FoV)
Partial Fourier or Half Fourier
Another way to speed up data acquisition is known as partial or half Fourier imaging [10]. In these techniques, the spacing between k-space lines is left unchanged, but some of the lines on one side of the center of k-space are not acquired. The reduction in scan time is directly proportional to the number of k-space lines left out of the acquisition. If, for example, only slightly more than half the k-space is acquired, the scan time can be reduced by approximately 50 %. Because each k-space line contributes to the overall imaging signal level, partial Fourier techniques result in a reduction in SNR. In practice, at least 5/8 of the data are collected to avoid SNR losses.
One way of reconstructing images from the resulting partial k-spaces is to simply insert zeros in place of the uncollected data, i.e., the technique known as zero-filling described in the previous section. The image quality obtained is similar to the fully-acquired image if the collected k-space fraction is close to one. However, a significant amount of blurring in the phase encoding direction occurs as this ratio approaches 0.5. An example of a partial Fourier acquisition where only 5/8 of the k-space has been acquired followed by a zero-filling reconstruction is illustrated in Fig. 5.5c.
In order to correct for this blurring, the sampled parts of k-space can be used to estimate the signal in the missing k-space parts in what is known as a homodyne reconstruction [11]; the symmetry properties of the k-space are exploited to synthesize the missing data after a correction is performed to account for any non-zero phase in the image, which can be caused by flow or motion. The homodyne reconstruction works well if the phase changes slowly over the image, but can lead to artifacts in areas with more rapidly changing phases. In these cases, the phase correction and the subsequent synthesis of uncollected data can be repeated in an iterative fashion. This algorithm, known as Projection Onto Convex Sets (POCS, [12]), reduces artifacts caused by rapidly changing image phase, and is often preferred over simple zero-filling or the homodyne reconstruction.
The partial Fourier technique is often used to reduce the turbo factor (TF), in single-shot TSE sequences [13]. By acquiring only slightly more than half of the k-space lines, the TF and therefore the acquisition time can be reduced by almost half. This technique, known as Half Fourier Acquisition Single-shot TSE (HASTE), enables acquisition times of less than 1 s per slice. However, the extremely long echo train leads to a significant T2 decay during the acquisition of one slice. This signal decay in turn causes considerable blurring in the reconstructed images, resulting in a deterioration of the spatial resolution. Therefore, HASTE is mainly used in cases where respiratory motion must be avoided and a low spatial resolution can be tolerated, for example to look at gross cardiac and vascular structures in the chest and abdomen.
Rectangular Field-of-View
In most standard views in cardiovascular imaging, such as the four chamber view, the dimensions of the patient’s body can be better described as a rectangle than as a square. In these cases, acquiring a square FoV would be a waste of acquisition time. Instead, the acquisition time can be shortened by using a rectangular FoV, in which the size of the FoV and matrix in the phase encoding direction are less than in the frequency encoding direction. By reducing the FoV in the phase encoding direction, fewer phase encoding lines are required, and the scan time is reduced compared to imaging with a square FoV. It should be noted that in order to maintain the image resolution, the highest phase encoding gradients should be left unchanged, but the distance between neighboring k-space lines increased instead.
View-Sharing
Another simple but powerful technique to accelerate dynamic MRI acquisitions is known as view-sharing. In a dynamic acquisition, such as the previously described cine imaging technique, it would be advantageous to keep the temporal resolution of each cine frame constant while doubling the total number of cine frames. In principle, this means that a larger number of k-spaces have to be “filled up” with acquired data. Instead of attempting to collect this additional data, which would generally not be possible if the acquisition window had been optimally selected, each of the available k-space lines is used for two consecutive cine frames, which is indicated in Fig. 5.6a. Instead of achieving a better temporal resolution, view-sharing can also be used to increase the spatial resolution or other imaging parameters. Although this technique involves the introduction of redundant information in the image reconstruction, the visual appearance of the cine movie generated with these data can be significantly improved due to the considerable increase in the reconstructed cine frames.
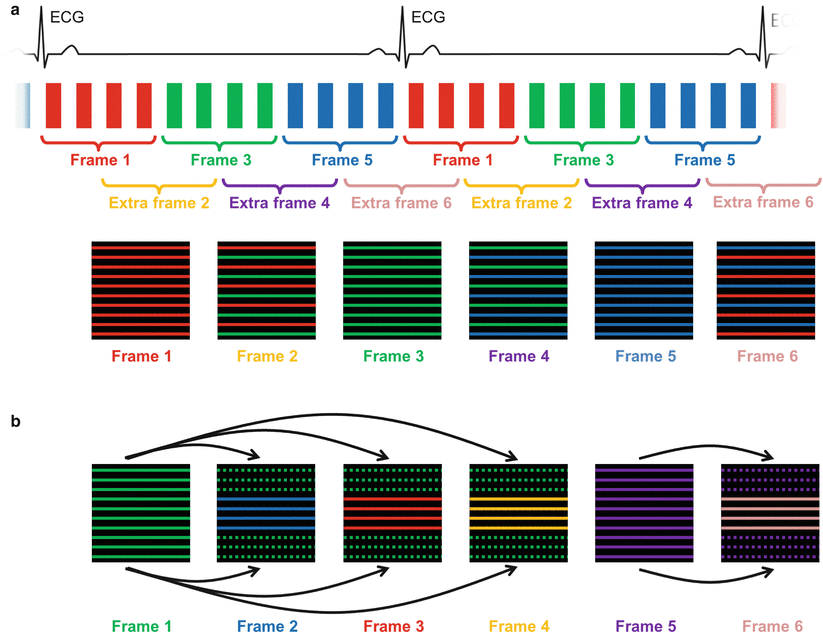
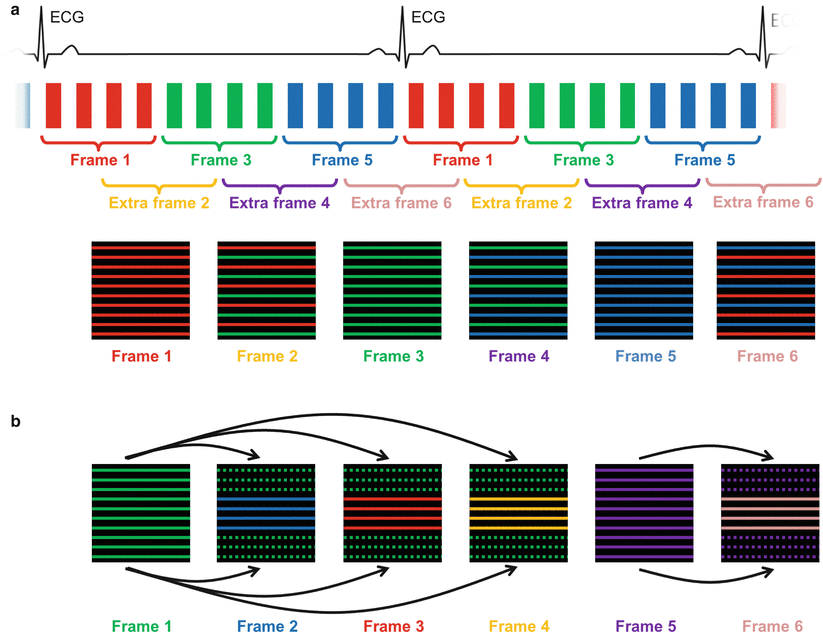
Fig. 5.6
View-sharing and keyhole techniques. In the view-sharing approach (a), each of the available k-space lines acquired in a dynamic image series is used for two consecutive cine frames. In this way, the apparent temporal resolution can be doubled without changing the spatial resolution. Keyhole imaging (b) can be used to accelerate the acquisition of a series of images where only the contrast changes over time. The assumed redundant information in the periphery of k-space is acquired infrequently (here only every fourth frame), while the contrast-relevant central parts of k-space are collected for each frame, leading to a high temporal resolution. The outer portions of k-space for these frames are filled using lines from the full acquisition. Because k-space lines are taken over a longer time frame, the temporal resolution for such an acquisition can be difficult to define
Keyhole Imaging
Another effective technique for the acceleration of dynamic acquisitions is the keyhole method [14, 15]. This technique is used to increase the temporal resolution of a series of images with changing contrast but similar features, such as contrast-enhanced MR angiography. In such datasets, it is assumed that most of the changes over time occur in the central portions of k-space, which contain the information about image contrast. The keyhole method works by acquiring only the central k-space lines for every frame, and filling up the rest of the k-space with a previously (or subsequently) acquired set of peripheral k-space lines. Because only the central lines are updated for each frame, and the outer lines are updated less frequently, the scan time for each frame is effectively reduced. The keyhole technique is illustrated in Fig. 5.6b. While keyhole methods can be used to obtain a higher temporal resolution without sacrificing spatial resolution, the longer temporal footprint of the entire dataset can lead to a blurring of temporal dynamics. If the outer lines of k-space are acquired significantly prior to or following the central lines of k-space, such that large image changes occur between the acquisitions, image artifacts can appear. For this reason, keyhole methods are only applicable in cases where the changes from image to image are minimal. Such methods are generally not used to capture motion but are limited to dynamic contrast changes, as in first-pass perfusion or dynamic angiography.
< div class='tao-gold-member'>
Only gold members can continue reading. Log In or Register a > to continue
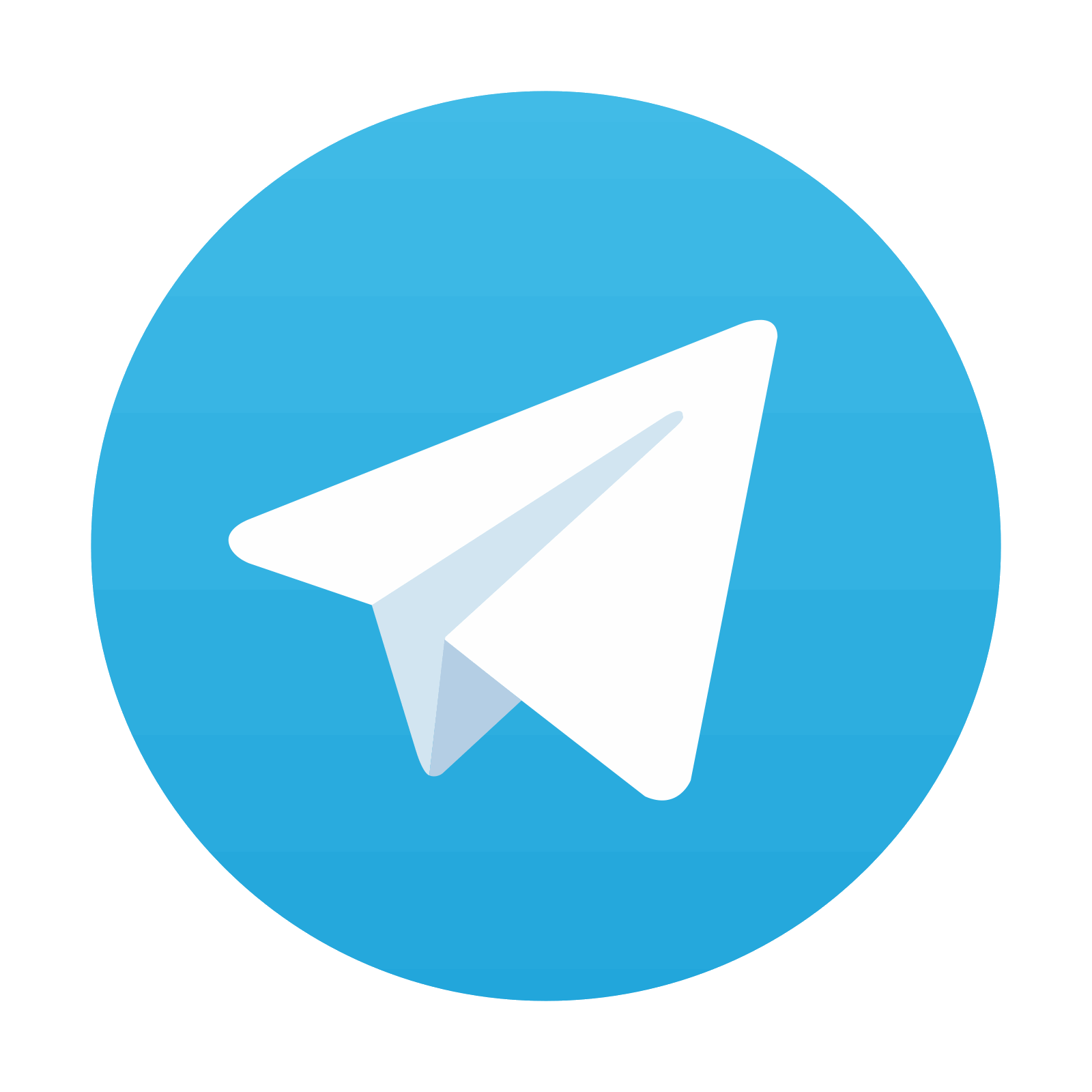
Stay updated, free articles. Join our Telegram channel
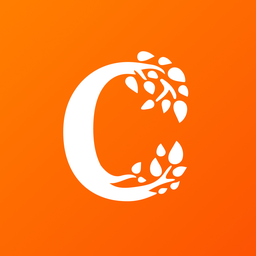
Full access? Get Clinical Tree
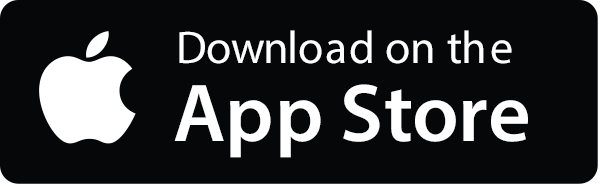
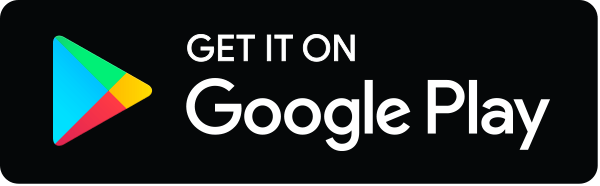