Chapter 10 Exercise Testing
Impaired exercise capacity can be defined by the inability of the subject to sustain a required work rate long enough for the successful completion of a given task that could be achieved by a healthy person. Limitation of oxygen transport from the atmosphere to the cell is the most common cause of exercise limitation in chronic respiratory disease, but altered oxygen utilization due to abnormally low mitochondrial oxidative potential also can be a contributing factor in patients with advanced disease (Figure 10-1).
Exercise Intensity and Energy Requirements
Alternative pathways of energy production not requiring oxygen constitute rapid but only transient solutions to fulfill the bioenergetic requirements. That is, anaerobic reduction of pyruvate to lactic acid in the cytosol is inefficient in terms of ATP production. Moreover, increased lactate levels provoke a marked fall in intracellular pH that may alter mitochondrial function. Likewise, skeletal muscle contains high levels of phosphocreatine (PCr) compared to other tissues, but the breakdown of muscle PCr stores can supply cellular needs of ATP for only a few seconds of strenuous contractions. Compared with glycolysis, aerobic metabolism requires longer to become activated. Energy utilization during exercise may require higher ATP turnover than the synthesis from aerobic pathways in the muscles allows. This phenomenon causes an “oxygen debt” that must be repaid after exercise. Aerobic metabolism, in turn, is more efficient in terms of ATP production and it enables the cell to utilize stored lipid as fuel via fatty acid metabolism. Consequently, the integrity of the different pathways governing cellular O2 transport–O2 utilization is a pivotal determinant of exercise capacity in both health and disease (see Figure 10-1).
Lactate threshold (LT), or anaerobic threshold, is the threshold for arterial lactate concentration increase. The LT should be considered to partition moderate from heavy-intensity exercise, and it corresponds to a transitional exercise intensity that triggers a series of physiologic responses associated with increased lactate production that stress ventilation, pulmonary gas exchange, and acid-base regulation. Of note, the LT is highly task-specific. It occurs at an appreciably lower
for arm exercise than for leg exercise and typically is lower for cycle ergometry than for treadmill exercise, reflecting the magnitude of muscle mass over which the work is distributed. A wide range of techniques have been advocated for estimation of LT, including both direct measurements and indirect estimation, as described next.
Critical power is the highest sustainable level of and usually is at an intensity below the maximal
for an individual subject and at an intensity of exercise that can still generate a plateau in
(Figure 10-2). Heavier exercise intensities, well beyond LT, are not sustainable and may peak at
max (see Figure 10-2). During moderate intensity submaximal exercise, the components of the O2 transport pathway can provide adequate O2 flux between the air and the mitochondria. Mitochondrial oxidative capacity will not have been reached, symptoms usually are tolerable, and muscle fatigue has not occurred, or at least may be insufficient to impair performance appreciably. Although the concept of critical power is relevant for understanding the exercise response, it is not assessed in clinical exercise testing, because no normal ranges for submaximal exercise comparable between laboratories or diseases have been established.
Maximal exercise (max) corresponds to maximal aerobic capacity, as displayed in Figure 10-2. At this point, maximal oxygen transport capacity and/or maximal mitochondrial oxidative potential have been reached. The subject cannot increase
when work rate is increased or the highest tolerated work rate is sustained for a period of time. Often,
max can only be clearly identified in fit subjects that can sustain high levels of exercise for a few minutes. Extreme motivation is needed to reach true
max, and it is, in general, an unsuitable and unsafe test for patient populations.
Peak exercise (peak) occurs when maximal work rate has been limited by severe symptoms at a level that does not require maximal O2 transport or maximal oxidative capacity. Under these conditions, a plateau in O2 (
max) has not been reached, and the appropriate designation is peak rather than maximal
. Symptom-limited exercise testing is common in the clinical setting, and this approach does not preclude adequate interpretation of the test results.
Because the catabolic capacity of the myosin ATPase is such that it outstrips by far the capacity of the respiratory system to deliver energy aerobically, exercise tolerance (max) is determined by the capacity of the O2 transport–O2 utilization system, rather than by the muscle’s contractile machinery (see Figure 10-1).
Responses to Exercise in Health and Disease
Pulmonary Response to Exercise in Lung Disease
In patients with COPD, resting levels of minute ventilation () are abnormally high but, during exercise, the slope between
and work rate is normal. For a given level of
during exercise, tidal volume (VT) tends to be lower and respiratory rate (f) higher in patients than in healthy subjects. Moreover, the O2 cost of breathing per unit ventilation is higher in persons with COPD than in healthy subjects. Impaired respiratory mechanics requires more effort to move a given volume of air. Peak exercise VT is strongly related to vital capacity in these patients, in whom two strategies are adopted during exercise to increase
: First, end-expiratory lung volume (EELV) increases, allowing higher maximum expiratory flow rates. This dynamic hyperinflation does not occur in normal persons, who show a fall in EELV during exercise (Figure 10-3). Second, inspiratory flow rate increases, so that inspiratory time decreases and more time is available for expiration.
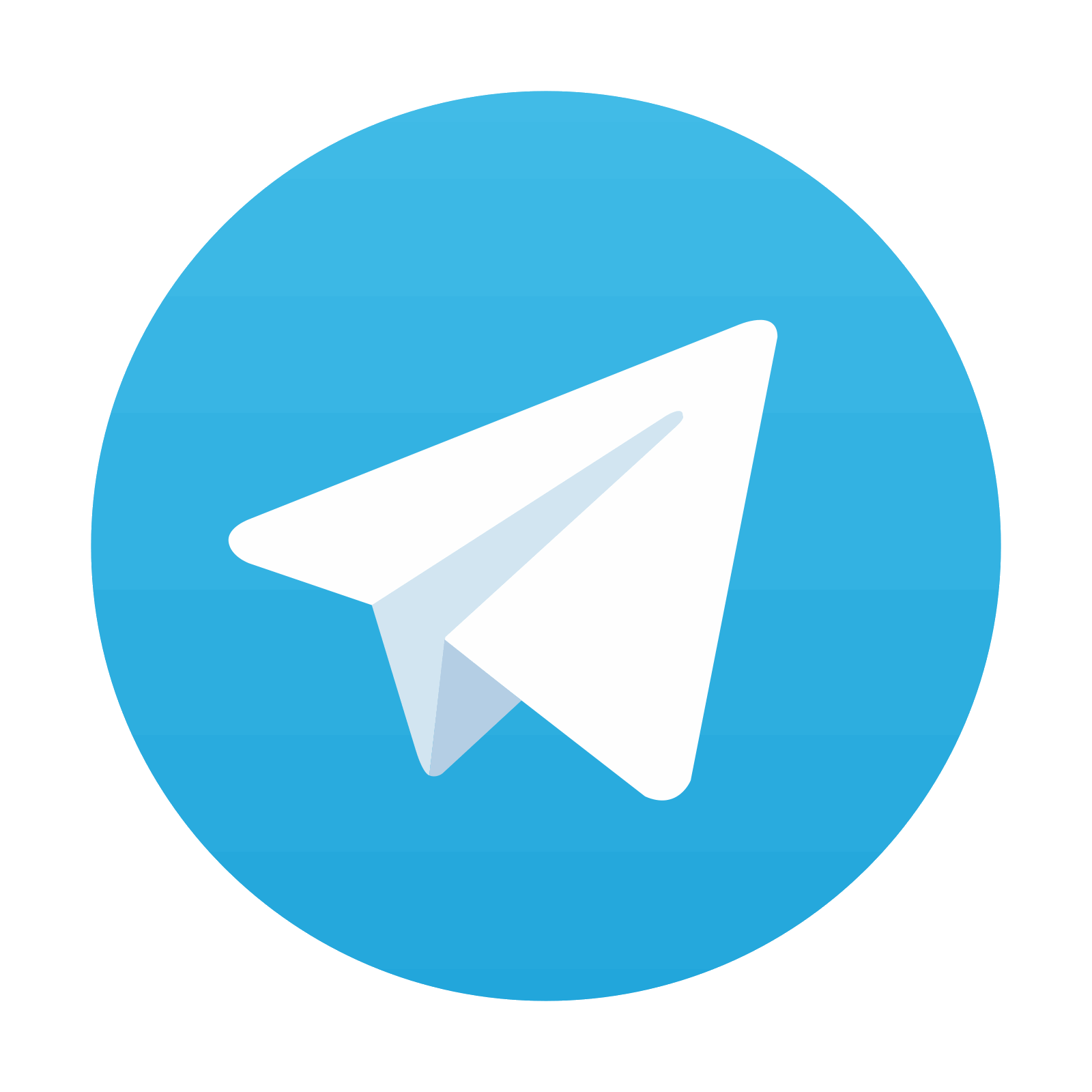
Stay updated, free articles. Join our Telegram channel
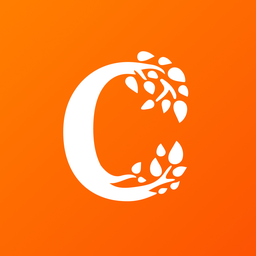
Full access? Get Clinical Tree
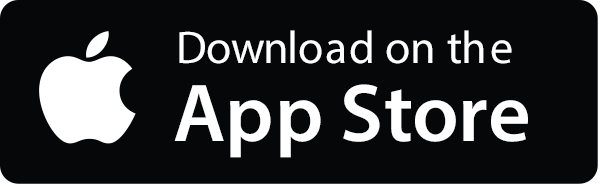
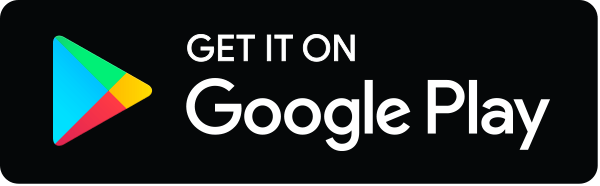